66 Urinary Levels of Organization
The primary function of the urinary system is to maintain homeostasis of fluid and small molecules within the body. We will start with a molecular characterization of urine. Then we will follow the movement of material from the bloodstream, through the functional units of the kidney through the other organs of the urinary system, and out of the body.
Molecular Level – The Components of Urine
Our input of water varies greatly from day to day. Despite major differences in fluid intake, the total volume of fluid within the body remains the relatively constant. The body’s fluid homeostasis is achieved largely due to the kidneys’ ability to regulate how much water is excreted in urine. If a person with healthy kidneys drinks a large volume of fluids, the kidneys will produce a large volume of urine. When a person with healthy kidneys does not drink enough fluids or experiences significant fluid loss, the kidneys will produce a small amount of concentrated urine to conserve water.
Urine is composed of urea, chloride, sodium, potassium, creatinine and other compounds (ions, inorganic and organic). Urea is an organic breakdown product of nitrogenous materials.
Characteristic | Normal Value/Nature |
---|---|
Color/transparency | Yellow/clear |
Odor | Varies from slightly aromatic to ammonia-like; food and beverages can change the odor |
pH | 4.5 to 8.0 (average: 6.0) |
Specific gravity (the density of urine compared with the density of pure water) | 1.001 to 1.035 |
Water content | 95 to 97 percent |
Volume | 1 to 2 liters/ day (quarts/day) |
Filtration, Reabsorption, and Secretion
To produce urine, nephrons and collecting ducts carry out three basic functions: glomerular filtration, tubular reabsorption, and tubular secretion.
The first step in urine production is glomerular filtration. In this process, water and most of the solutes in blood plasma pass through the wall of glomerular capillaries, first into the glomerular capsule and then into the renal tubule. The product of glomerular filtration is referred to as glomerular filtrate.
During tubular reabsorption, cells of the tubule reabsorb almost all water and a variety of solutes from the filtrate as it flows through the renal tubule and collecting duct. These reabsorbed substances are then returned to the blood in the peritubular capillaries and vasa recta. In contrast to absorption, in which new substances enter the body (e.g., through the gastrointestinal tract), reabsorption refers to substances that have been removed from the blood being returned to the blood.
In the process of tubular secretion, renal tubule and collecting duct actively transport substances (e.g., wastes, drugs, excess ions) from the blood in the peritubular capillaries. Secretion, in this case, refers to the removal of substances from the blood. In contrast, the secretion of hormones or enzymes, for example, refers to cells releasing substances into blood, ducts, and interstitial fluid.
The normal amount of filtrate produced as a result of glomerular filtration is so high that the amount of fluid entering the proximal convoluted tubules in 30 minutes is greater than the total blood plasma volume. Clearly, some of this fluid must be reclaimed and sent back into the bloodstream to maintain water balance and ion concentrations. This reclamation process occurs through reabsorption in the tubules. Secretion is essentially the opposite of reabsorption. It removes the additional solutes from the plasma and actively transports them to the tubule. Many students get confused initially with this concept that secretion means end up in the urine and reabsorption means ends up back in the blood.

Glomerular Filtration
High Pressure Bulk Filtration
The glomerular capillaries filter about one fifth of the plasma that flows through the kidneys into the renal tubules. Glomerular filtration works like any other filtration process. For example, a coffee filter prevents large coffee grounds from passing through it, while allowing the passage of water and small solutes such as flavor molecules and caffeine. In the same way, the glomerular filtration membrane blocks the passage of blood cells and proteins, while allowing water and other solutes from the blood to pass into the glomerular capsule. These substances are forced through the membrane by the higher hydrostatic pressure in the glomerular capillary than in the capsular space.
The glomerulus has the highest rate of filtration of any capillary bed in the body for a number of reasons. First, its filtration membrane has a very large surface area. In addition, the large fenestrations (capillary pores) in glomerular capillaries make them at least 50 times more permeable to water and solutes than other capillary beds. Finally, the blood pressure (hydrostatic pressure) in glomerular capillaries is about three times higher than in other capillary beds (around 55 mm Hg versus 18 mm Hg or less). This increased blood pressure is due to the relatively small pressure drop across the afferent arteriole leading into the glomerular capillary and the large pressure drop across the efferent arteriole, which leads out of the glomerular capillary. Looking at filtrate production, the combined daily output of all other capillary beds in the body is about three to four liters (three to four quarts). In the kidneys, the daily output is 150 liters (158 quarts) in women and 180 liters (190 quarts) in men. Tubular reabsorption returns more than 99 percent of the glomerular filtrate (the fluid than enters the capsular space) to the bloodstream. This means than just one to two liters are eliminated in urine. The filtration fraction describes the portion of blood plasma in the afferent arterioles that ends up as glomerular filtrate. A typical filtration fraction ranges from 16 to 20 percent.
The Filtration Membrane
The porous filtration membrane separates the inside of the glomerular capsule from the blood. Water and solutes smaller than about three nanometers in diameter (e.g., glucose, amino acids, nitrogenous wastes) pass freely across the membrane between the blood and capsule. This means there are similar concentrations of these substances in both the blood and the glomerular filtrate. Some bigger molecules may find a way through the membrane, but molecules larger than five nanometers (large proteins and blood cells) do not usually get into the tubule.
The filtration membrane, three layers serve as barriers to larger molecules circulating in the blood. From most permissive to most restrictive, these layers are the glomerular capillary endothelium, the gel-like basal lamina basement membrane, and the podocyte-formed filtration slit. All plasma components (note plasma does not include blood cells) can pass through the fenestrations in the endothelium, but blood cells cannot. The smallest proteins and most other solutes can penetrate the basement membrane. This membrane is composed primarily of negatively charged glycoproteins that oppose other macromolecular anions and obstruct their entry into the tubule. In other words, the basement provides electrical selectivity to the filtration process. Most plasma proteins are too large to meet the size restrictions. Moreover, because most plasma proteins have a net negative charge, they are repelled by the negatively charged glycoproteins of the basement membrane. Any macromolecules that do manage to pass through the basement membrane face yet another barrier, thin membranes called slit diaphragms that span the filtration slits. Specialized cells in the glomerulus, known as mesangial cells, will destroy macromolecules that are trapped in the filtration membrane. Mesangial cells can also contract and alter the capillary surface area available for filtration.
Net Filtration Pressure
Three forces affect glomerular filtration rate (volume filtered per unit time); hydrostatic pressure of the blood in the glomerulus, hydrostatic pressure of the fluid in the capsular space, and colloid osmotic force of the blood in the glomerulus. Hydrostatic pressure within the glomerular capillaries promotes or enhances filtration. The other two forces oppose filtration and are: back pressure from hydrostatic pressure of the fluid within the capsule and colloid osmotic pressure caused by the plasma proteins within the glomerular capillaries. Subtracting the opposing pressures from the promoting pressure yields the net filtration pressure.
Hydrostatic Pressure – Resistance from fluid in the tubule – Osmotic Collodial Pressure = Net Filtration Pressure
Pressure | Description | Average | Effect |
---|---|---|---|
Glomerular hydrostatic | Glomerular capillary blood pressure | 55 mm Hg | Promotes filtration |
Capsular hydrostatic | Hydrostatic pressure applied to the filtration membrane by fluid in the capsular space and renal tubule | 15 mmHg | Opposes filtration |
Blood colloid osmotic | Results from proteins present in blood plasma | 30 mmHg | Opposes filtration |
The glomerular hydrostatic pressure (i.e., the blood pressure in glomerular capillaries) is the primary force responsible for pushing water and solutes from blood across the filtration membrane. This unusually high pressure (55 mm Hg) is opposed by two forces that resist the influx of fluids: the capsular hydrostatic pressure exerted by fluid already in the glomerular capsule and the blood colloid osmotic pressure caused by proteins present in blood plasma (e.g., albumen, fibrinogen). Net filtration pressure averages 10 mm Hg (i.e., 55 mmHg −15 mmHg − 30 mmHg = 10 mmHg). This is in contrast to the 0.3 mmHg net pressure found in most capillaries of the body.
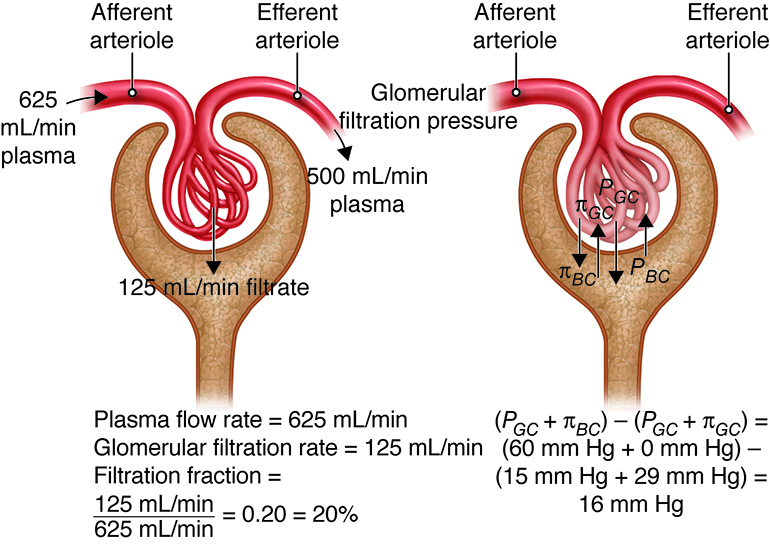
The glomerular filtration rate (GFR) is the total amount of filtrate formed by the two million renal corpuscles in the kidneys divided by time. The average GFR is 125 milliliters (4 ounces) per minute, which adds up to nearly 140 liters (50 gallons) a day. The kidneys must maintain a relatively consistent GFR to prevent homeostatic imbalances in body fluids. If the GFR is too high, needed substances will rush through the renal tubules too quickly to be completely absorbed, and they will be lost in urine. A GFR that is too low will allow waste products to accumulate in the plasma, ultimately leading to illness and death if not corrected.
Tubular Reabsorption
Reabsorption starts as soon as filtrate enters the proximal convoluted tubules. Before the reabsorbed substances can reenter the blood, they must pass through three barriers: the luminal and basolateral membranes of cells in the tubule, and the endothelium of the peritubular capillaries.
Reabsorption returns about 99 percent of filtered water and many filtered solutes to the bloodstream. Although cells in the proximal convoluted tubule perform most reabsorption, epithelial cells all along the length of the renal tubule and the collecting duct also contribute to this process. To maintain normal plasma levels, almost every organic nutrient (e.g., glucose, amino acids) is completely reabsorbed. The amount of water and number of ions reabsorbed is determined by hormonal signals and is based on homeostatic needs. The scope of reabsorption can be appreciated by looking at water. Each day, about 180 liters (190 quarts) of water are filtered in the kidneys. Only 1–2 liters (1–2 quarts) are excreted in urine; the rest is reabsorbed in the kidneys.
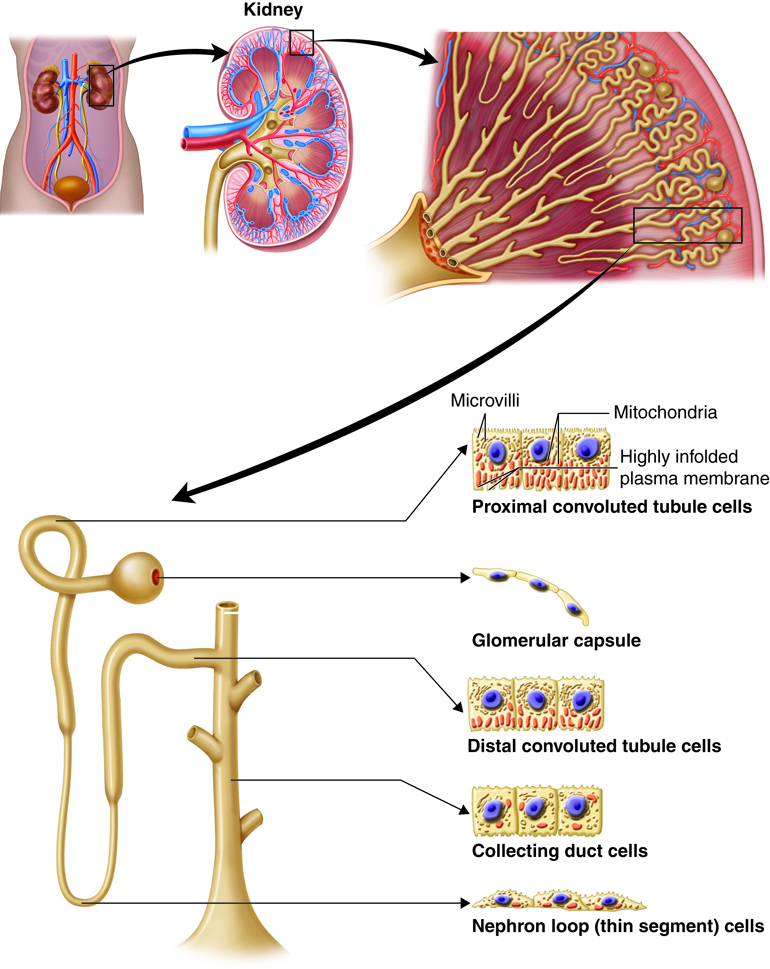
In the table below, review the partial list of substances filtered through the kidneys. As you can see, for most of these substances very little is excreted in the urine. For example, only .03 grams of the 275 filtered grams of Bicarbonate ions are excreted daily while none of the glucose that is filtered through the kidneys is excreted. On the other hand, a larger proportion of the filtered Urea is excreted when compared to the other substances.
Substance | Filtered* (grams/day) | Reabsorbed (grams/day) | Excreted in Urine (grams/day) |
---|---|---|---|
Bicarbonate ions | 275 | 274.97 | 0.03 |
Chloride ions | 640 | 633.7 | 6.3 |
Creatinine | 1.6 | 0 | 1.6 |
Glucose | 162 | 162 | 0 |
Potassium ions | 29.6 | 29.6 | 2.0** |
Proteins | 2.0 | 1.9 | 0.1 |
Sodium ions | 579 | 575 | 4 |
Urea | 54 | 24 | 30*** |
Uric acid | 8.5 | 7.7 | 0.8 |
It is not necessary to memorize the numbers, but note the importance of reabsorption.
- *Refers to the amount entering the glomerular capsule, assuming GFR is 180 liters (190 quarts) per day.
- **After almost all filtered potassium ions are reabsorbed in the convoluted tubules and nephron loop, principal cells in the collecting duct secrete some of these ions.
- ***Urea is also secreted (in addition to being reabsorbed and excreted).
Reabsorbed substances can be returned to the bloodstream to maintain homeostasis by going between tubule cells (paracellular reabsorption) or by going through individual cells (transcellular reabsorption) on their way to entering a peritubular capillary. Cells in the renal tubule are joined together at tight junctions. Although these junctions are tight, they are just leaky enough to let some solutes pass through during paracellular reabsorption. Substances reabsorbed through the transcellular route move through the tubule cell’s apical membrane, then through the intracellular fluid, and finally through the basolateral membrane to reach the interstitial fluid. The apical membrane is in contact with the tubular fluid, and the basolateral membrane is in contact with interstitial fluid as well as the bottom and sides of cells.
The apical and basolateral membranes of renal cells contain a variety of transport proteins. The mechanism of transport depends on the substance being reabsorbed. In passive reabsorption, no ATP is required. In active reabsorption, the energy derived from the hydrolysis of ATP helps “pump” the substance across a membrane—either directly or indirectly—during at least one step in the reabsorption process.
Because all water is reabsorbed via osmosis, the reabsorption of water is coupled with the reabsorption of solutes. About 90 percent of water reabsorbed in the kidneys is accompanied by the reabsorption of sodium ions, chloride ions, glucose, and other solutes. Because the water is “obliged” to tag along with the reabsorbed solutes, this process is called obligatory water reabsorption. It occurs in the water-permeable proximal convoluted tubule and descending limb of the nephron loop. The remaining 10 percent of water is reabsorbed through facultative water reabsorption, which is controlled by antidiuretic hormone and takes place primarily in the collecting ducts.
When filtrate enters the proximal convoluted tubule, it is referred to as tubular fluid or tubular filtrate. The composition of the tubular fluid changes as it travels through the tubule and collecting duct, as each segment reabsorbs different substances.
Reabsorption in the Proximal Convoluted Tubule
The majority of solute and water reabsorption occurs in the proximal convoluted tubule. Sodium ions, which are the most plentiful cation (positively charged ion) in the tubular fluid, participate in most solute reabsorption. Sodium ion carriers co-transport all filtered glucose, amino acids, lactic acid, water-soluble vitamins, and other nutrients. The reabsorption of sodium ions into the interstitial fluid and then into the blood creates the osmotic gradient needed for water reabsorption. An osmotic gradient is produced when the solute concentration in the interstitial fluid is higher than that in the tubular fluid.
Region | Reabsorbed Substances | Amount Absorbed(Percent) | Mechanism |
---|---|---|---|
Proximal convoluted tubule | Water | 65 | Obligatory water reabsorption |
Sodium ions (Na+) | 65 | Primary active transport | |
Potassium ions (K+) | 65 | Passive transport; paracellular route | |
Glucose, amino acids, and most other organic solutes | 100 | Secondary active transport with Na+ | |
Chloride ions (Cl−) | 50 | Passive transport; paracellular diffusion | |
Bicarbonate ions (HCO3−) | 80-90 | Secondary active transport with Na+ | |
Urea | 50 | Passive diffusion | |
Calcium ions (Ca2+) | variable* | Passive transport; paracellular route | |
Magnesium ions (Mg2+) | variable* | Passive transport; paracellular route |
*Amount reabsorbed depends on the needs of the body.
Reabsorption in the Nephron Loop
Because all glucose, amino acids, and other nutrients are reabsorbed in the proximal convoluted tubule, fluid flows into the nephron loop (loop of Henle) at a rate of 40 to 45 milliliters per minute (mL/min) (1.5 ounces/min), down from 125 mL/min (4.5 ounces/min) in the proximal convoluted tubule. The chemical composition of the tubular filtrate at this point is very different from that of the glomerular filtrate even though the osmolarity is similar. The fluid’s osmolarity is still comparable to that of blood, because water was reabsorbed as solutes were reabsorbed throughout the proximal convoluted tubule. Since part of the nephron loop is comparatively water-impermeable, the reabsorption of water in this region is not necessarily linked to the reabsorption of solutes. Water is reabsorbed by osmosis in the water-permeable descending limb, but not in the ascending limb. This water reabsorption in the water-permeable descending limb can occur because the descending limb dips down toward, or into, the renal medulla, where the osmolarity of the interstitial fluid increases. This creates a gradient for this water reabsorption to occur. Essentially no solutes are reabsorbed in the descending limb, while solutes are reabsorbed via both active and passive mechanisms in the ascending limb. The permeability differences in these two limbs plays an important role in the ability of the kidneys to form either dilute or concentrated urine.
Region | Reabsorbed Substances | Amount Absorbed(Percent) | Mechanism |
---|---|---|---|
Descending limb | Water | 15 | Obligatory water reabsorption |
Ascending limb | Na+ | 20–30 | Secondary active transport; paracellular diffusion |
K+ | 20–30 | Secondary active transport; paracellular diffusion | |
Cl− | 35 | Secondary active transport; paracellular diffusion | |
HCO3− | 10–20 | Secondary active transport with Na+ | |
Ca2+ | variable* | Passive paracellular diffusion | |
Mg2+ | variable* | Passive paracellular diffusion |
*Amount reabsorbed depends on the needs of the body.
Reabsorption in the Distal Tubule and Collecting Duct
Because 80 percent of filtered water is reabsorbed by the time tubular filtrate enters the distal convoluted tubules, the flow of this fluid has slowed to a rate of approximately 25 mL/min (1 ounce/min). Most reabsorption in this segment occurs in the initial portion of the distal convoluted tubule. When the fluid reaches the end of the distal tubule, 90 to 95 percent of solutes and water have been reabsorbed. Both principal and intercalated cells located here and in the collecting duct. Principal cells reabsorb sodium ions (and secrete potassium ions), while the intercalated cells reabsorb potassium ions and bicarbonate ions (and secrete hydrogen ions). The needs of the body determine how much of these solutes, and of water, are reabsorbed in the late distal convoluted tubule and collecting duct.
Region | Reabsorbed Substances | Amount Absorbed(Percent) | Mechanism |
---|---|---|---|
Distal convoluted tubule | Water | 10–15 | Obligatory water reabsorption |
Na+ | 5 | active Na+ transport | |
Cl− | 5 | active Na+ transport | |
Collecting duct | |||
Water | variable* | Facultative water reabsorption; antidiuretic hormone required to insert aquaporins (water channels) | |
Na+ | 3 | Primary active transport (requires aldosterone) | |
Na+, H+, HCO3−, Cl−, hydrogen ions (H+) | variable* | Primary active transport of Na+ and the medullary gradient create the conditions for passive transport of some HCO3− and Cl− and co-transport of H+, Cl−, and HCO3− | |
K+ | variable* | K+ is both reabsorbed and secreted (aldosterone dependent), usually resulting in net K+ secretion | |
Urea | variable* | Facilitated diffusion in response to concentration gradient in the deep medulla region; recycles and contributes to medullary osmotic gradient |
*Amount reabsorbed depends on the needs of the body.
Tubular Secretion
There are two methods of removing unwanted substances from filtrate. First, tubular cells simply do not reabsorb some solutes. The second method is tubular secretion. Secretion transfers unwanted substances from the blood and tubule cells into the tubular fluid. With the exception of potassium ions, most secretion occurs in the proximal convoluted tubule. However, some secretion also occurs in the cortical regions of the collecting ducts and in the late distal convoluted tubule.
Tubular secretion is important for a number of reasons. It removes substances that are not easily filtered, such as some drugs and metabolites that are securely attached to plasma proteins. Second, it disposes of unwanted substances or end products that have been reabsorbed passively, such as urea and uric acid. Third, it eliminates excess potassium ions. Finally, it regulates blood pH. As an example, when blood pH becomes too acidic, secretion adds more hydrogen ions (acid) into the filtrate and retains more bicarbonate ions (a base).
Secretion in the Proximal Convoluted Tubule
With the exception of potassium ions, the proximal convoluted tubule is the primary site of secretion. This tubule secretes a range of organic ions, including hydrogen ions and ammonium ions. Ammonium ions are a poisonous waste product of deamination (the process that removes an amino group from an amino acid). Urea is also secreted in this tubule.
Secretion in the Distal Convoluted Tubule and Collecting Duct
Secretion and reabsorption of sodium, potassium, hydrogen, and bicarbonate ions are regulated and adjusted depending on various circumstances. For instance, the rate of potassium ion secretion is adjusted for the dietary intake of potassium, with the goal of maintaining a stable potassium ion level in body fluids. Potassium ions are regularly being brought into principal cells by basolateral sodium-potassium pumps. This process results in a high intracellular concentration of potassium ions. There are leakage channels in the apical (luminal) membrane of principal cells through which sodium ions enter and potassium ions escape. This mechanism of secretion is the primary source of the potassium ions that are excreted in the urine.
Summary of Tubular Reabsorption and Secretion
Reabsorption removes useful substances from the glomerular filtrate and returns them to the blood. The proximal convoluted tubule is the primary site of absorption; it is where all glucose, most amino acids, and the majority of salts are reabsorbed. Water reabsorption is coupled with the reabsorption of solutes in the proximal convoluted tubule in a process called obligatory water reabsorption. Only about 10 percent of water is reabsorbed in the collecting ducts, with the help of antidiuretic hormone, in a process called facultative water reabsorption. Secretion removes unwanted substances from the filtrate, such as urea and uric acid, so they can be excreted in urine. Secretion also helps regulate blood pH by adjusting the hydrogen ion and bicarbonate ion content of the filtrate. Several hormones affect reabsorption and secretion.
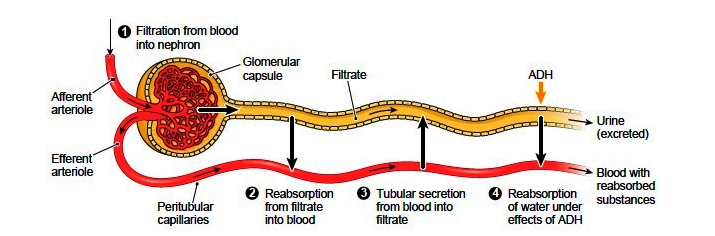
Urine Formation
The process of osmosis plays a major role in the regulation of urine concentration. Recall that osmosis refers to the flow of a liquid across a semipermeable membrane to equalize the solute concentration on both sides of the membrane. The ability of the solution to cause osmosis, its osmotic activity, depends on the amount and not the type of solute particles that cannot pass through the semipermeable membrane. For example, in the same volume of water, the osmotic activity of 10 sodium ions is the same as that of 10 glucose molecules, and the same as 10 proteins, and so on. Thus, the osmotic activity of a solution is based on the osmolarity of that solution, a quality defined as the number of solute particles dissolved in one kilogram of water. Osmotic activity is frequently measured in units of 0.001 mole of particles, a millismol (mOsm). Osmolarity is measured in mOsm/liter.
Formation of Dilute Urine
The ratio of water and solutes in glomerular filtrate is the same as that in blood, with an osmolarity of around 300 mOsm/liter (one mOsm is translated as a milli osmole or one thousandth of an osmole). The osmolarity of the filtrate that enters the proximal convoluted tubule is still the same. And when filtrate enters the nephron loop’s descending limb, its osmolarity is the same as both blood plasma and cortical interstitial fluid. Because tubular fluid is already dilute as it flows through the ascending limb of Henle’s loop, the formation of dilute urine is a relatively simple process. When the hypothalamus (via the posterior pituitary gland) is not secreting ADH, additional water is not reabsorbed in the collecting ducts and dilute urine is produced. Before urine enters the renal pelvis, it is further diluted by the removal of ions, including sodium ions, in the distal convoluted tubule and collecting duct. As a result, the osmolarity of urine can be reduced to nearly one fourth of that in the glomerular filtrate or plasma, dropping to as low as 70 mOsm.
Formation of Concentrated Urine
If a person does not drink enough water, sweats a lot, or has diarrhea, water conservation is required. In such cases, the kidneys will produce a small amount of concentrated urine to preserve water, while still eliminating wastes and surplus ions. Antidiuretic hormone (ADH), as its name suggests, inhibits diuresis (urine production). ADH helps the kidneys generate urine that is up to four times as concentrated as glomerular filtrate or blood plasma (up to 1,200 mOsm/liter for urine versus 300 mOsm/liter for glomerular filtrate). ADH can do this only when there is an osmotic gradient of solutes in the renal medulla’s interstitial fluid, in other words, when the solute concentration in the interstitial fluid is higher than that in the tubular fluid. This osmotic gradient, coupled with the insertion of aquaporins into the luminal membrane of the collecting ducts’ principal cells, causes water and urea to pass from the filtrate into the interstitial space to equalize osmolarity. At maximum ADH secretion, as much as 99 percent of the water in the tubular filtrate is reabsorbed, and the kidneys produce about half a liter of highly concentrated urine per day. The production of concentrated urine is key to our ability to survive for an extended period of time without water.
Countercurrent Flow
The osmotic gradient needed to produce concentrated urine depends on two chief factors. First, individual areas of the nephron loop differ in permeability and reabsorption characteristics. Second, fluid flows in opposite directions through adjacent tubes in different parts of the urinary system. This process is called countercurrent flow of fluid. It occurs down and up the descending and ascending limbs of the nephron loop. Blood flowing along the ascending and descending portions of the vasa recta also follows countercurrent flow. Two countercurrent mechanisms operate in the kidneys: countercurrent multiplication and countercurrent exchange.
Countercurrent Exchange
In countercurrent exchange, countercurrent flow enables the passive exchange of water and solutes between blood in the vasa recta and the medullary interstitial fluid. The osmolarity of blood entering the vasa recta is around 300 mOsm/liter. In its descending limb, urea and sodium and chloride ions diffuse from the increasingly concentrated medullary interstitial fluid into the blood, while water diffuses from the blood into the interstitial fluid. Along the ascending limb of the vasa recta, the concentration of the interstitial fluid steadily decreases. At this point, urea and sodium and chloride ions diffuse from the blood back into the interstitial fluid, and water diffuses in the opposite direction. Because the osmolarity of blood leaving the vasa recta is only a little higher than that of blood leaving it, it can provide oxygen and nutrients to the renal medulla without washing out the osmotic gradient. To summarize, the osmotic gradient in the renal medulla is created by countercurrent multiplication in the nephron loop, and it is maintained by countercurrent exchange in the vasa recta.
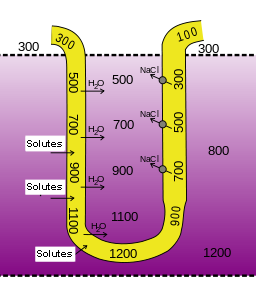
Countercurrent Multiplication
The nephron loop is responsible for the countercurrent multiplication mechanism that establishes an osmotic gradient within the medullary interstitium. This gradient is necessary for the collecting duct to create an osmotic gradient.
Three factors are involved in countercurrent multiplication. First, recall that the ascending limb is responsible for the active transport of sodium, chloride and potassium out of the tubular fluid and into the interstitium. Because this limb is relatively water impermeable, the solutes move into the interstitial fluid without additional water, increasing the osmotic pressure of the interstitium.
Second, the descending limb, which is in very close proximity to the ascending limb, is solute-impermeable and water-permeable. Because the ascending and descending limb share the same interstitial fluid, water moves along the osmotic gradient from the descending limb into the interstium, concentrating the tubular filtrate in the descending limb. Thus, the osmolarity of the medullary interstitial fluid along this limb steadily increases, and water leaves the filtrate. The osmolarity of the filtrate is highest (1,200 mOsm) where the nephron loop bends, and the filtrate moves from the descending limb to the ascending limb.
The third factor in the countercurrent multiplication is the shift of the fluid along the length of the tubule so that fluid that participated in water loss in the descending limb, will now participate in solute loss in the ascending limb. When the filtrate in the descending limb turns the corner and enters the ascending limb, the concentrations of sodium and chloride ions are very high in the filtrate The molecules are now actively pumped from the tubule into the interstitial fluid to maintain the high osmotic pressure in the interstitium. It turns out that this system “traps” high salt concentrations deep in the medulla as the ions are most actively pumped out there.
Losing salt but not water makes the filtrate in the ascending limb increasingly more dilute. By the time it reaches the distal tubule, it is at 100 mOsm and therefore has a lower osmotic pressure than the blood plasma and interstitial fluids in the renal cortex. This is what allows for the generation of a dilute urine.
Another contributor to the high osmolarity of the the medullary interstitium is the recycling of urea. Urea from the interstitial fluid diffuses into the filtrate in the thin limbs of the nephron loop. Because the thick limb and collecting duct are urea-impermeable, by the time the filtrate reaches the collecting duct, water reabsorption has created highly concentrated urea that diffuses back into the medullary interstitial fluid. The resulting pool of urea is a major contributor to the high osmolarity in this region. This urea is then recycled back into the thin limbs of the loop, and the cycle starts again. The presence of ADH amplifies urea recycling, which, in turn, amplifies the osmotic gradient and enables the formation of more concentrated urine.
Urine Transport, Storage, and Elimination
Urine Transport
The difference in hydrostatic pressure between the glomerular capsule (10 mm Hg) and the renal pelvis (practically no pressure at all) creates a pressure gradient that forces filtrate to flow from the glomerular capsule through the tubules and into the renal pelvis. In contrast, no pressure gradient exists to propel the flow of urine through the ureters and into the urinary bladder. Instead, urine flow at this point is controlled by peristaltic contractions in the circular smooth muscle of the ureter walls. These peristaltic waves vary in frequency from once every few seconds to once every two to three minutes. Their frequency is increased by parasympathetic stimulation and decreased by sympathetic stimulation.
Kidney stones are one of the most painful urinary system disorders. Also called calculi (“little stones”), kidney stones are not a new disorder. Scientists have discovered them in 7,000-year-old Egyptian mummies. Kidney stones are formed when the uric acid salts, magnesium, or calcium in urine crystallize. Certain types of kidney stones run in families and appear to have a hereditary component. Some substances in foods may also contribute to the incidence of kidney stones. Most calculi are small enough to pass through the urinary tract and be eliminated with urine. Stones larger than five millimeters in diameter, however, can prevent urine from draining. The backed-up urine exerts increasing pressure in the kidney, causing extreme pain in the back and side near the kidney or in the lower abdomen. Dehydration can also contribute to the formation of kidney stones. Each year, about 2.4 million Americans seek treatment for kidney stones. Kidney stones are more common in people with a family history of calculi, or who have urine retention or frequent bacterial urinary tract infections. The most commonly used treatment for kidney stones is a procedure called extracorporeal shock wave lithotripsy. Shock waves created outside the body pass through the skin and body tissues and break down the stones into small particles that can be eliminated in the urine. In severe cases, endoscopic or open surgery may be required to remove the stones.
Urine Storage
Much more time is spent storing urine than micturating (urinating). When urine accumulates in the bladder, distension of the bladder walls activate stretch receptors. These receptors transmit signals through visceral afferent fibers to the sacral area of the spinal cord. The signals initiate spinal storage reflexes, which enhance sympathetic inhibition of the bladder’s detrusor muscle and maintain the internal sphincter in a closed position. The reflexes also stimulate pudendal motor fibers, which cause the external urethral sphincter to contract, preventing the urine from escaping.
Urine Elimination
As previously noted, micturition (also called urination or voiding) is the process of emptying urine from the bladder and is the result of involuntary and voluntary muscle contractions. When the amount of urine accumulated in the bladder reaches about 200 milliliters (7 ounces) (though this amount varies from person to person), afferent impulses are sent to the sacral region of the spinal cord that initiate a reflexive relaxation of the internal urethral sphincter and contraction of the detrussor muscle. The afferent signals that stimulate the urge to urinate are also sent to the brain. This allows the person to relax their external uretheral sphincter, made of skeletal muscle, so that micturition will occur. If we do not void immediately, the reflexive responses will initially weaken, but as more volume is added to the bladder, these responses will come back more strongly, creating a more urgent need to void.
When we are ready to empty the bladder—a decision executed by the cerebral cortex—the micturition reflex is set in motion. Afferent impulses activate the micturition center of the brain. This signal intregrates with parasympathetic signals of the spinal cord to allow the external sphincter to relax, thus releasing urine from the bladder.
If we need to delay micturiition, the reflex bladder contractions will taper off and stop within about one minute, and urine will continue accumulating. The addition of another 200 to 300 milliliters (7 to 10 ounces) of urine will prompt another micturition reflex. If voiding is still not possible, the reflexes will again subside. When more than 500 to 600 milliliters (about 20 ounces) of urine accumulates, urination will occur whether we want to or not. After micturition, about 10 milliliters (0.33 ounces) of urine will remain in the bladder.
Summary of Filtration, Reabsorption, and Secretion
Despite major differences in fluid intake on a given day, the total volume of fluid within the body usually remains the same. This is due to homeostasis. For example, if a person with healthy kidneys drinks a large volume of fluids, the kidneys will produce a large volume of urine. Conversely, if that same person does not drink enough fluids, the kidneys will produce a small amount of concentrated urine to preserve water.
- Urine is composed of elements and compounds that include urea, chloride, sodium, potassium, creatinine, hydrogen, and bicarbonate. The nephrons help produce urine.
- Nephrons and collecting ducts carry out three basic functions in urine production:
- glomerular filtration where water and solutes in blood plasma pass through the wall of glomerular capillaries and filter into the renal tubules about one fifth of the plasma that flows through the kidneys.
- tubular reabsorption where cells of the tubule reabsorb almost all water some solutes from the filtrate as it flows through the renal tubule and collecting duct. These reabsorbed substances are then returned to the blood via the peritubular capillaries and vasa recta.
- tubular secretion where cells in the renal tubule and collecting duct secrete waste from the blood as it flows through the tubule and duct.
- Three forces affect the rate of glomerular filtration; one enhances filtration, and two resist it. Subtracting the opposing pressures from the promoting pressure yields the net filtration pressure.
- Hydrostatic Pressure – Resistance from fluid in the tubule – Osmotic Collodial Pressure = Net Filtration Pressure
- The glomerular filtration rate (GFR) is the total amount of filtrate formed by the two million renal corpuscles in the kidneys divided by time.
- The average GFR is 125 milliliters (4 ounces) per minute, which adds up to nearly 140 liters (50 gallons) a day.
- The kidneys must maintain a relatively consistent GFR to maintain homeostatic balance in body fluids.
- Reclaimed fluid that is sent back into the bloodstream to maintain water balance and ion concentrations occurs through reabsorption in the tubules. Reabsorption returns about 99 percent of filtered water and many filtered solutes to the bloodstream.
- Each day, about 180 liters (190 quarts) of water are filtered in the kidneys. Only 1–2 liters (1–2 quarts) are excreted in urine; the rest is reabsorbed in the kidneys.
- Reabsorption is performed by cells in the proximal convoluted tubule, epithelial cells all along the length of the renal tubule, and the collecting duct.
- The majority of solute and water reabsorption occurs in the proximal convoluted tubule and sodium ions participate in most solute reabsorption.
- Secretion removes the additional solutes from the plasma and actively transports them to the tubule.
- Tubular secretion occurs mostly in the proximal convoluted tubule and removes unwanted substances from filtrate by transferring unwanted substances from the blood and tubule cells into the tubular fluid.
- Tubular secretion is important because:
- It removes substances that are not easily filtered
- It disposes of unwanted substances or end products, such as urea, that have been passively reabsorbed.
- It eliminates excess potassium ions.
- It regulates blood pH.
- In the urinary system, osmosis plays a major role in the regulation of urine concentration.
- Osmotic activity is the ability of the solution to cause osmosis and depends on the amount of solute particles that cannot pass through the semipermeable membrane.
- Osmolarity is defined as the number of solute particles dissolved in one kilogram of water. The osmotic activity of a solution is based on the osmolarity of that solution.
- Formation of Dilute and Concentrated Urine:
- Dilute urine is produced when the pituitary gland is not secreting the antidiuretic hormone (ADH)and the diluted filtrate goes to the renal pelvis.
- When water conservation is required, the kidneys will produce a small amount of concentrated urine to preserve water, while still eliminating wastes and surplus ions. ADH inhibits urine production.
- Micturition is the process of urination
- Which begins with blood carrying various wastes enters each of the two kidneys through the renal artery.
- From there, afferent impulses are sent to the sacral region of the spinal cord when the amount of urine in the bladder reaches about 200 milliliters.
- Once afferent impulses are perceived, a reflexive relaxation of the internal urethral sphincter is initiated and contraction of the detrussor muscle occurs.
- When more than 500 to 600 milliliters (about 20 ounces) of urine accumulate in the bladder, involuntary urination will occur.
- Micturition ends when urine exits out of the body through the urethra.
- After micturition, about 10 milliliters (0.33 ounces) of urine will remain in the bladder.