29 Skeletal Levels of Organization
The skeletal system is organized into the following structural hierarchy, from microstructure to gross anatomy:
- Chemistry and molecular scale – Water, minerals, collagen, and other proteins.
- Nanoscale – Mineral crystals are embedded within collagen to form composite fibers.
- Submicron scale – Mineralized fibrils are organized into a lamella (plural is “lamellae”).
- Micron (micrometer) scale – Lamellae form osteons in compact bone or trabeculae in spongy bone.
- Macroscale – Compact and spongy bone combine together to form whole bones, and bones combine at articulations to form the skeletal system.
Bones constantly store and release calcium, phosphate, proteins, and other matrix components of bone. Within the matrix, the mineral crystals resist compression but are very brittle. The organic components, such as the collagen fibers and the cells, help give the bone some flexibility. The hierarchical organization of the skeletal system at different scales is important for its mechanical, biological, and chemical functions.
On a chemical level minerals such as calcium and phosphate form crystals that are able to resist compression of the bones. However, by themselves, these minerals can make the bones brittle. These crystals can be embedded within the macromolecules, mainly collagen, which add flexibility to the bone. The cellular level of bone includes osteoblasts, osteoclasts and osteocytes, which build bone, resorb bone and sense forces, respectively. Together the minerals, macromolecules and cells for a tissue that provides structural integrity, responds to its surroundings, and stores minerals.
Molecules and Macromolecules
The major components of bone tissue at the molecular scale are minerals, water, collagen, and other proteins. At the next level of organization, small crystals of hydroxyapatite made of calcium and phosphate are embedded within collagen fibers to produce a composite (blended) material with high compressive and tensile strength.
The skeletal system protects and supports vital organs, allows our body to move, stores important minerals, and produces blood cells. There are several chemical elements and molecules required to maintain the many functions of the skeletal system. The chemical properties of these components support bone structure and function. On a chemical level, bone is divided into inorganic and organic (carbon-containing) components.
The primary inorganic components of bone are:
- calcium, which is required for many functions throughout the body;
- phosphorus (in the form of phosphate ions), which is a component of buffer systems and energy-rich molecules; and
- water, which contributes to the compressive resistance of bone and contributes to the fluid matrix of bone.
The primary organic components of bone are:
- collagen, the major structural protein (type I in bone and type II in cartilage); and
- proteoglycans, which are negatively charged glycosylated proteins (glycosylated means having carbohydrate sugar groups modifying the protein).
Bone is approximately 60 to 70 percent inorganic mineral and 10 percent water by weight. The remaining 20 to 30 percent of bone is organic matrix (osteoid), such as collagen and proteoglycans. Your body contains 1 to 2 kilograms of calcium and nearly 600 grams of phosphorous. Nearly 99 percent of the calcium and 86 percent of the phosphorous is stored in your bones.
Components of Bone
Inorganic Components of Bone
Calcium ions (Ca2+) are stored in bone tissue, but can be released into the bloodstream when blood levels fall below optimal. Blood calcium is important for muscle contractions, nerve impulses, and blood clotting.
In bone, phosphorous (P) is found in the form of phosphate ions (H2PO4–). Outside of bone, phosphorous plays roles in energy storage (such as in ATP), and is required for the formation of DNA and RNA. Therefore, it is required for cellular growth, maintenance, and tissue repair.
When combined with hydrogen, phosphorous forms dihydrogen phosphate ions (H2PO4–). Dihydrogen phosphate acts as a buffer to maintain a constant pH balance by acting as either a hydrogen ion donor (acid) or a hydrogen ion acceptor (base). In all cells, a constant pH must be maintained to carry out cell functions.
As mentioned in previous sections, hydroxyapatite is an inorganic calcium phosphate mineral that is a primary component of bone. Crystal hydroxyapatite has the chemical formula: Ca10(PO4)6(OH)2.
During bone formation, the collagen fiber matrix is formed. Next, mineralization of the matrix occurs when calcium, phosphate, and water from the extracellular fluids combine to form insoluble hydroxyapatite. The hydroxyapatite incorporates into the small pores within collagen fibrils and crystallizes into long, thin, nanosized plates within the collagen network. The incorporation of hydroxyapatite within the collagen fibers contributes to the overall compressive strength of bone. Because the crystals are nanosized, they have a large surface area. The large surface area of the crystals makes it easy to release ions into the extracellular fluids when blood levels decrease.
Sometimes bones contain fluoride. Fluoride is similar to the hydroxyl ion in charge and size. When fluoride replaces the hydroxyl ion in hydroxyapatite crystals, it forms fluoroapatite. Fluoroapatite is a more compact and less soluble crystal than hydroxyapatite. Therefore, ion exchange at the crystal surface is slower. The primary role of fluoride in bone is to strengthen bone mineral.
Water is an important molecular component of bone matrix. Water is initially present in the spaces within the collagen matrix of the unmineralized bone extracellular matrix (osteoid). As crystals fill in the spaces between collagen fibrils, the bone is mineralized and the water is displaced. The water that remains in bone after this process is complete forms hydrogen bonds with polar, hydrophilic components of collagen. Water also assists with the compressive resistance of bone due to osmotic interactions caused by negatively charged proteoglycans.
In addition to calcium, phosphorous, water, and fluoride, the inorganic parts of bone also contain sodium, potassium, carbonate, and magnesium. Although these molecules do not form their own crystals, they are bound to hydroxyapatite crystals within bone mineral.
Organic Components of Bone
You can think of the hydroxyapatite in bones as being similar to concrete. The chemical structure of hydroxyapatite gives it a high mechanical strength under compression (squeezing force), but little strength under tension (pulling force). If our bones were made only of hydroxyapatite, they would be very fragile, like concrete. They would break easily under tension. The organic components of bone help to give it additional strength and flexibility.
Collagen, a ropelike, fibrous protein, is the major organic component of bone. Like rope, collagen has significant mechanical strength under tension. Therefore, collagen provides tensile strength to the bones. The spiral arrangement of collagen in combination with its ECM (extracellular matrix) components, such as water and specialized proteins, also makes bone more elastic and less brittle, so that it can absorb shock more easily.
Bone matrix is a network of collagen fibers and hydroxyapatite. It is strong under both tension and compression. Furthermore, the collagen contributes to its ability to absorb sudden forces without breaking.
Collagens are a class of fibrous proteins found in bone, cartilage, and other connective tissues. The primary structure of collagen has repeats of glycine-proline-X, where X can be any other amino acid. This repeated arrangement gives collagen a unique helical secondary structure. The tertiary structure of collagen is a triple helix fibril, which further twists into a quaternary structure of a collagen fiber. The resulting “twisted, twisted, twist” is similar to the higher-ordered structures in rope.
Type I collagen is the primary form of collagen found in bone. The collagen fibers in bone are approximately 300 nanometers long, and are arranged in a staggered pattern. During bone formation, the collagen fiber matrix is deposited first. Proteoglycans are a second class of organic components found in bone. Proteoglycans are macromolecules composed of proteins with negatively charged glycosylated modifications. There are a number of proteoglycans, which are classified by the type of side chains on the core protein. The side chains on the proteoglycans are linear carbohydrates. As with cartilage, the presence of the negatively charged proteoglycans contributes to the resilience of the tissue and retention of water within the matrix. Proteoglycans also bind and release molecules for cell signaling.
Organic and Inorganic Combination
Mineralization of the bone matrix occurs when calcium, phosphate, and water from the extracellular fluids combine to form insoluble hydroxyapatite. The hydroxyapatite incorporates into the small pores within collagen fibrils and crystallizes into long, thin, nanosized plates within the collagen network. The incorporation of hydroxyapatite within the collagen fibers contributes to the overall compressive strength of bone. Within the bone matrix, the minerals, water, collagens, and proteoglycans function together to provide strength in tension and compression. Additionally, this unique environment binds and releases chemicals into the extracellular fluids for use throughout the body.
Example: Osteogenesis Imperfecta
Osteogenesis imperfecta (from osteo–, meaning bone, and genesis, meaning production or beginning) is a congenital (genetic) disorder that affects the structure and strength of bones. The condition is primarily caused by genetic mutations that affect the structure and/or quantity of type I collagen produced by the body. Remember that collagen is an important component of the extracellular matrix of bone tissue. In people with osteogenesis imperfecta, the lack of collagen (or presence of defective collagen) prevents the extracellular matrix from forming correctly. The mineral structure of the bone is weakened, and as a result, the bones are unusually brittle.
The bones of people with osteogenesis imperfecta break extremely easily, sometimes with only small amounts of pressure. The bones’ brittleness reduces their ability to support and protect the body. They also typically take longer to heal and may be more prone to infection.
Bones become stronger and thicker when they are exposed to regular stress. Conversely, bones that do not experience regular stress may lose extracellular matrix and become less dense. In a person with osteogenesis imperfecta, bones that break often (and therefore spend a lot of time immobilized in casts or restraints) are exposed to much less stress. As a result, they may lose even more extracellular matrix and become even more brittle.
Extracellular Matrix in Bone
You have already learned about the hydroxyapatite and collagen combination, which is responsible for giving bones their rigidity. The skeletal system is an excellent example of a system that is primarily composed of extracellular matrix. Generally, cells of the body function within a matrix to hold them together. In the case of bone, the extracellular matrix (ECM) is composed of crystals of hydroxyapatite and long collagen proteins. The hydroxyapatite and collagen work together to give bone its unique properties of strength, compressive resistance, and flexibility.
The previous section described the importance of the bone matrix components calcium and hydroxyapatite in maintaining bone rigidity. However, bone remodeling is equally important to maintaining bone health. Throughout your lifetime, your bones are constantly remodeled and repaired. There are three main cell types in bone, which maintain bone balance and strength:
- osteoclasts, which break down and reabsorb bone;
- osteoblasts, which deposit and build new bone; and
- osteocytes, which are mature bone cells that act as sensors for repair.
Think of the bones of your body as a rapidly expanding city. When the old buildings get outdated, they are either remodeled to make enhancements or knocked down to make room for the new ones. As soon as a building is knocked down, a new one is erected in its place. The construction crew recycles the old building materials. Many city planners constantly monitor the building progress and talk with one another about which sections of the city will get the available resources.
Osteoclasts secrete an acid that dissolves the inorganic components of bone: calcium and phosphate. Osteoclasts also contain enzymes that digest the organic components of the bone matrix, such as the proteins and proteoglycans. Bone resorption (osteolysis) is complete when small cavities remain on the bone surface. Osteoclast activity is important for creating the medullary cavities of diaphyses, which house bone marrow. (Remember that the diaphysis is the main shaft of a long bone.) Osteoclasts are important for removing calcium from bones if the blood calcium levels fall, such as when a diet is deficient in calcium. Remember calcium is critical for life and if levels fall, it could be fatal if it wasn’t taken from the bones.
Osteoblasts build bone and fill in the cavities created by osteoclasts. Osteoblast cells secrete osteoid (the organic portion of the bone matrix) around them, and then mineralize the matrix to generate rigid bone tissue. Bone mineralization occurs through osteoblast secretion of an enzyme called alkaline phosphatase. Alkaline phosphatase cleaves phosphate groups to provide the necessary phosphate for building hydroxyapatite. Osteoblasts are stimulated when blood calcium levels increase. The excess calcium is deposited into the bones for storage.
After bone mineralization, osteoblasts trapped within the matrix differentiate into osteocytes. Osteocytes have long processes (outgrowths or protrusions) for connection to other osteocytes within the bone. This arrangement allows osteocytes to act as mechanical sensors. Osteocytes sense mechanical strain within bone and regulate the activity of osteoblasts or osteoclasts, depending on needs for calcium and phosphate. To maintain bone strength, bone resorption and bone deposition must be in constant balance with each other.
Macroscopic Structures of the Skeletal System
You have learned that bone tissue is classified into two types based on structure: compact bone and spongy bone. The parallel arrays of lamellae are organized into different arrangements depending on the bone structure. The lamellae in compact bone form tubular structures, called osteons. The osteons of compact bone are oriented in the direction of the load-bearing axis. The osteons also create a central canal for the passage of blood vessels. Osteocytes in the osteons are embedded in small cavities called lacunae (singular is lacuna), and are oriented around the central canal parallel with the lamellae on the load-bearing axis. The diaphyses of long bones are stronger on their long axis than in any other direction, because of the parallel array of osteons and osteocytes.
The lamellae in spongy bone form a random mesh-like structure of interconnecting plates called trabeculae. Likewise, osteocytes within spongy bone are randomly arranged. The strongest trabeculae in spongy bone are arranged on the bone axis that undergoes the most stress. Flat bones of the skull are primarily made of spongy bone and are good at resisting forces from many directions because of the trabecular arrangement.
In both types of bone tissue, the mineral components, calcium and phosphate, combine with collagen to provide the compressive and tensile strength of bone. Spongy and compact bone tissues are combined to create bones, which store and release calcium and phosphate into the blood through constant resorption and deposition. Many bones then articulate with each other to form the skeletal system.
Ossification Process and Bone Repair Mechanisms
Bones form in two ways. A process known as intramembranous ossification forms bones that develop from layers of connective tissue. Flat bones such as those found in the skull develop through this process. Endochondral ossification (from the word roots endo-, meaning “within,” and chondral, meaning “cartilage”) is bone formation from a hyaline cartilage blueprint or template, which determines the future bone shape. Bones of the limbs and extremities develop through endochondral ossification. For example, an infant’s arm and leg bones contain only small amounts of actual hard bone material; they are primarily made of cartilage. As the child grows, bone replaces the cartilage.
Ossification is the process of forming bone. You learned that there are two types of ossification:
- intramembranous ossification, which is direct synthesis of bone by specialized stem cells (mesenchymal cells) from fibrous connective tissue; and
- endochondral ossification, which is synthesis of bone from a (hyaline) cartilage template.
Intramembranous Ossification
Intramembranous ossification is the process that forms and repairs the flat bones of the skull, clavicles and other irregularly shaped bones. In some situations of bone repair and adaptation to excessive force, intramembranous ossification generates new bone.
The process of intramembranous ossification involves multiple steps:
- Increased vascularization.
- Recruitment of mesenchymal stem cells
- Differentiation
- Secretion of osteoid
- Mineralization
- Formation of trabeculae
The site for future bone formation increases in vascularization—new blood vessels form near the site where the bones will grow. Mesenchymal stem cells, which originate in the embryonic mesoderm, become active and travel through the blood vessels to the future site of bone formation. Chemical messages then cause the mesenchymal stem cells to differentiate: they change into osteoprogenitor cells, which may divide and differentiate into osteoblasts. The osteoblasts deposit osteoid (the unmineralized bone extracellular matrix) and are then trapped in the matrix, where they differentiate into osteocytes. Inorganic salts in the blood travel through the blood vessels to mineralize the bone matrix. As a result, hydroxyapatite crystals form within the osteoid. On the interior of the tissue, small clusters of bone begin to connect with other clusters to form trabeculae. Osteoblasts near the surface of the bone deposit matrix in organized lamellae and form a thin outer layer of compact bone. The periosteum (“peri-” means “surrounding” and “osteum” means “bone”) is living membrane composed of fibrous connective tissue that forms on the outside of the compact bone. Its inside layer has osteoblasts for bone growth and repair.
Endochondral Ossification
Most bones of the skeleton below the skull develop through endochondral ossification.
This process involves the following steps:
- Formation of a cartilage template
- Growth of the template
- Differentiation
- Vascularization
- Calcification
- Bone formation
The first step is formation of a hyaline cartilage template, which is the shape of the desired new bone. The cartilage template grows in size and thickens through the production of new chondroblasts at the perichondrium. The perichondrium is the cartilage equivalent of the periosteum. Chondroblasts differentiate into chondrocytes, which produce chemical messages that stimulate the increase of vascular supply at the perichondrium. This increase in vascular supply brings in inorganic salts, which mineralize the central cartilage matrix.
Cartilage is laid down as a template that provides some mechanical stability. This is like when designers and architects build a template out of balsa wood, clay or foam because it is easy to quickly remodel and manipulate those substances. Then, once the template is worked out, they will remodel it using a stronger material. In bone, the ‘model’ cartilage is remodeled over time and osteoblasts produce a full bone matrix with new collagen and hydroxyapatite. In this way, biology works more efficiently than any engineered tissue graft.
Bone Mechanics, Formation, and Aging
After bone formation, bone resorption and bone deposition occur continually in a process called bone remodeling to allow for skeletal response to mechanical use, nutritional status and as part of the bone repair and healing process. In the absence of malnutrition or disease, this process maintains homeostasis of both total bone mass and inorganic substances such as calcium and phosphate.
As bones age, they tend to decrease in density and, as a consequence, decrease in strength. In some people, especially women, the bones become very brittle and easily broken. The result is a disorder called osteoporosis. Within bones affected by osteoporosis, bone mass and mineral content decrease. As a result, the bones develop canals filled with fibrous and fatty tissues. This leads to an increased risk of bone fracture because the bone organization that is important to weight bearing is lost.
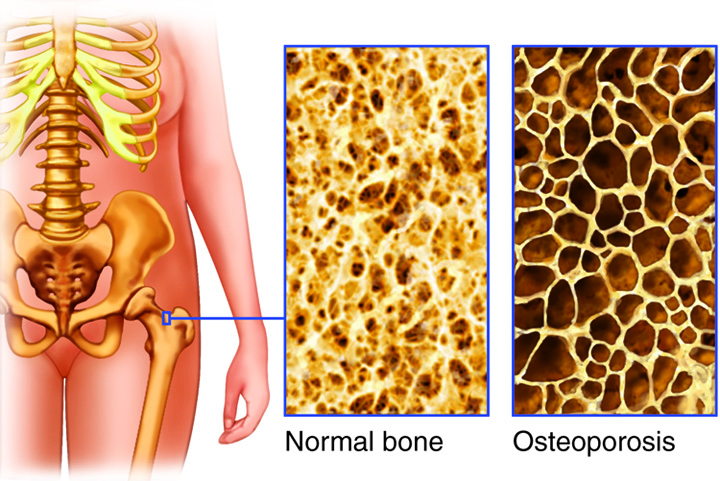
Fractures
The microscale structure of a bone gives it significant strength and rigidity, but extreme forces can cause bones to break, or fracture. The table below describes the most common types of fractures.
Type of fracture | Description |
---|---|
Simple fracture (also called a closed fracture) | A fracture in which a bone breaks completely, but the broken ends do not penetrate the skin |
Compound fracture (also called an open fracture) | A fracture in which the ends of the broken bone break through the skin |
Comminuted fracture | A fracture in which the bone is shattered or broken into several pieces |
Greenstick fracture | A fracture that occurs in children when one side of a bone breaks, and the other side bends |
Impacted fracture | A fracture in which one end of the fractured bone is forced into the interior of the other end |
Stress fracture | A set of tiny fractures or fissures in a bone caused by repeated stress on the bone |
A bone fracture affects the bone on several levels of organization. A fracture involves a physical break in the mineral structure of the bone. Fractures typically cause blood vessels in the bone to rupture, reducing the blood flow to the bone tissue. As a result, some of the cells in the surrounding bone die. These dead cells and the related cellular debris are removed by immune cells and osteoclasts. Over time, various cell types—fibroblasts, chondroblasts, and osteoblasts—work together to repair the mineralized bone tissue.
Fractures weaken bone, making it less able to perform its functions of support and protection, although once healed, the site of the fracture is stronger than the remaining bone. A fracture may cause a change in the shape of the bone. If that happens, then the way the bone responds to a contracted muscle may change. As a result, fractures can prevent bones from moving correctly when muscles pull on them.
There are pain receptors and nerves in the bone. The pain experienced when a fractured bone moves is one way the body reacts to help itself heal. Bones heal more quickly and thoroughly if they are kept immobilized (which is why the typical treatment for a broken bone is to put it in a cast or other restraint). Because we instinctively avoid actions that cause pain, the pain that occurs when a broken bone is moved causes us to minimize the movement of that bone. This, in turn, helps keep the bone stable while it heals.
Severe fractures, such as compound fractures or comminuted fractures, can cause long-term or permanent disruptions to the body’s homeostasis. Because a compound fracture breaks through the skin, bacteria and other pathogens can enter the body after a compound fracture. Those pathogens can enter the bone, blood, muscles, or other tissues or organs, causing severe infection. Severe fractures are also less likely to heal correctly without medical (typically surgical) intervention. Improperly healed fractures can cause changes in the bone’s reaction to force, leading to changes in body motion (such as a limp).