40 Nervous Sensory Functions
Recall that the nervous system often plays important roles in homeostasis, including our ability to respond to both our internal and external environment. In order to respond and maintain homeostasis, we must be able to detect that a change has happened in the first place. The nervous system is well adapted to carry out this specific function, by converting various internal and external stimuli into electrical signals in the form of post-synaptic potentials or action potentials. Any action potentials generated are then carried along neurons, and various forms of electrical signals at synapses and neural networks are integrated for decision making purposes. Not all stimuli are used only for homeostasic maintenance, with sensory input playing a role in all kinds of reflex and conscious responses as well.
In this section we will look more closely at how the nervous system converts physical and chemical signals such as touch, taste, light, sound, and others into electrical signals that can be interpreted and processed by the central nervous system in a manner that allows us to perceive them. This overall process of sensing and interpreting these signals is called sensation. For the purposes of study, sensation is typically divided into general and special senses. Regardless of whether it is a general or special sense, the signal is received by receptors that then convey information to the CNS.
Types of Receptors
Sensory receptors enable us to learn about the environment around us or about the state of our internal environment. Stimuli from varying sources, and of different types, must be received and changed into the electrochemical signals of the nervous system represented by changes in the membrane potential. The sensory information is relayed to the central nervous system where it is integrated with other sensory information, or sometimes higher cognitive functions, to become a conscious perception of that stimulus. The central integration may then lead to a motor response.
Sensory Receptors
Stimuli in the environment activate specialized receptors in the peripheral nervous system. The classification of receptors into types can be based on three different criteria: structure of the receptors, location of the receptors relative to the stimuli they sense, and by the types of stimuli to which they respond. Regardless of type, the function of these receptors is to transduce a stimulus from one form of energy (chemical, physical, etc.) into a change in the cell membrane potential that may or may not create an action potential.
Structural Receptor Types
The cells that detect a change in the environment can be neurons with free nerve endings, where the dendrites are exposed to the surrounding tissue; neurons with encapsulated endings, where supporting cells aid in the reception of stimuli; or specialized receptor cells, which have specific structural components for detecting stimuli. Examples of neurons with free nerve endings are the pain and temperature receptors in the dermis of the skin. Also in the dermis are encapsulated nerve endings such as the lamellar corpuscle that senses pressure. The cells in the retina that receive light stimuli are an example of specialized photoreceptor cells, not neurons, that in turn can stimulate an associated sensory neuron.
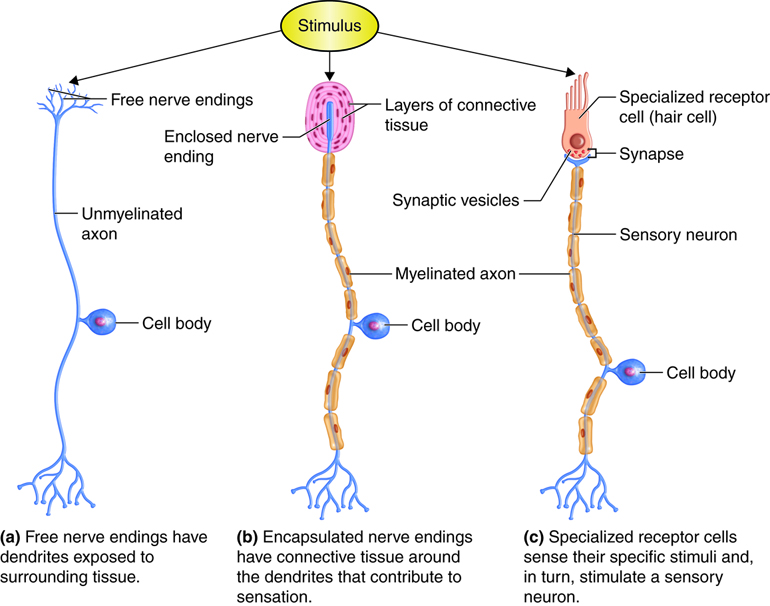
Receptors can also be classified based on their location relative to the stimuli. Exteroceptors are receptors that receive input from the external environment, such as the lameller corpuscles of the dermis and photoreceptors of the eye that have already been mentioned. Interoceptors are those that sense stimuli from the internal organs. Examples would include a stretch receptor in the wall of an organ, such as those that sense the increase in blood pressure in the aorta or carotid artery or detects stretch as the bladder fills with urine. Finally, proprioceptors are widely distributed receptors in muscles, tendons and joint capsules that the body uses to determine position and movement of structures, such as its limbs and fingers. Proprioceptors allow you to touch your finger to your nose, even with your eyes closed.
Functional Receptor Types
Lastly, receptors can be classified by the types of signals they transduce into changes in membrane potential.
Chemoreceptors sense chemical stimuli, examples being taste, smell and the osmotic pressure of the body’s extracellular fluids (the latter sensed by osmoreceptors).
Nociceptors are pain receptors. Although pain is primarily a chemical sense that detects the presence of chemicals released during tissue damage, nociceptors are typically considered in a functional category of their own. Nociceptors are found in most tissues throughout the body, exceptions being the brain and possibly certain internal structures of organs.
Mechanoreceptors sense physical stimuli, such as pressure and vibration, as well as the sensation of sound and pull of gravity. A specific example of a mechanoreceptor is the baroreceptors (pressure receptors) found in the carotid arteries, which sense blood pressure.
Thermoreceptors are specific to sensing temperature and changes in temperature. Thermoreceptors are found in two forms, those that respond most strongly to temperatures below normal body temperature (cold thermoreceptors), and those that respond most strongly at temperatures above normal body temperature (warm thermoreceptors). At normal body temperature, both types of receptors are active, but there is generally no awareness of cold or warmth.
Photoreceptors respond to electromagnetic radiation (light). Humans have the ability to sense electromagnetic waves at wavelengths between 400 and 700 nanometers, with different wavelengths corresponding to different colors.
Introduction to the General (Somesthetic) Senses
Because the central nervous system requires a significant amount of input in order to carry out homeostatic functions, receptors are numerous. Some of these receptors are widely distributed throughout body tissues (general or somesthetic senses), while others are localized to special sense organs of the head, such as the eye or ear (special senses). Collectively, the receptors and associated neurons that sense and process information related to our somesthetic senses are called the somatosensory system. We will first review the general categories of receptors found in this system, along with their distribution.

The widely distributed receptors of the somesthetic senses can be classified based on the anatomical structures in which they are located. They are termed somatic senses when they are located in, and sense information from, the somatic structures of skin, muscles, joints (including the related structures of tendons and ligaments) and organ capsules. The somesthetic senses also include visceral senses, the ability to sense the chemical environment of our blood and body fluids. Visceral senses also include monitoring the “state” of internal organs (ex. pressure and stretch receptors in vessels and in organs other than skin, muscles and joints). Visceral senses are particularly important to the function of the autonomic nervous system, whose function is to maintain many of these variables near a set point.
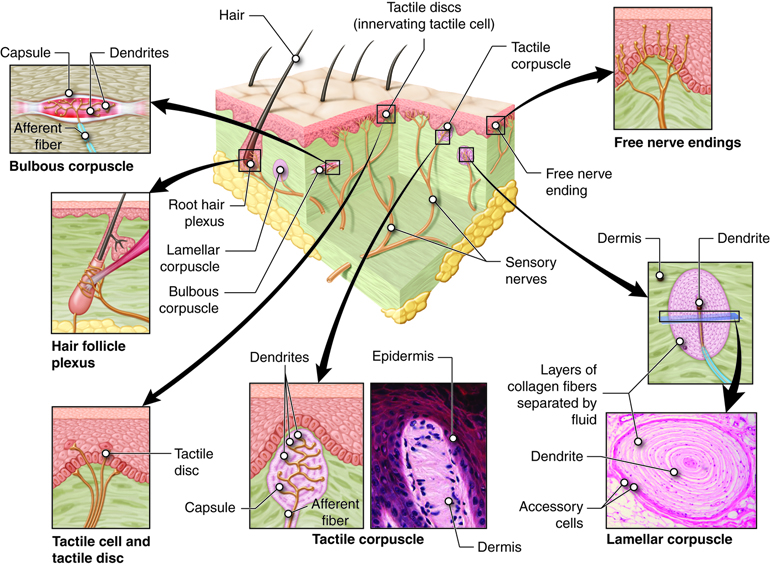
Within an organ, receptors will be found in various places. For example, in the skin, there are sensory receptors in both the epidermis and dermis; and in skeletal muscles, sensory receptors are found embedded in the contractile elements as well as in the tendons.
Sensory Modalities and Adaptation
The receptors that provide information for somesthetic senses come in a variety of anatomical and functional types. Each specific receptor will respond to only one type of functional signal (such as mechanical or chemical, but not both). If the information is transmitted all the way to the brain’s cortex, we perceive a sensation. The type of perception that this information leads to is called a modality. There are four main modalities typically recognized as part of the somesthetic senses. These include temperature, touch, pain (nociception), and position and movement (proprioception). Yet each of these can be further subdivided into sub-modalities (sometimes called stimulus modalities). For example, the modality of pain can be subdivided into sharp, dull and aching.
There is not general agreement over how sensory modalities should be categorized and subcategorized. In some classification schemes the general sense of touch is replaced with pressure. In others, there may be differences in the subcategories of touch. For example, there is evidence that a tickle occurs with simultaneous activation of certain touch and pain receptors – as long as other conditions are appropriate. As you are probably aware, others can tickle you, but you generally cannot tickle yourself, even if you can activate the same sensory receptors. Thus input from other systems seems to be able to affect our sensations. There is still a lot for scientists to learn about sensory perception.
Sensory Adaptation
When first jumping into cool water, you may endure a wave of sensory information “reminding you” that the water is cool. Yet minutes later, you may be “used to” the water temperature, or have adapted to it. This change in perception did not occur because the water warmed up, but because the sensory receptors that originally responded to the change in temperature are no longer sending signals to the CNS, or are sending them at a decreased rate. This example indicates how a rapidly adapting receptor might function; it provides significant signals to the CNS about the original change in temperature that the body experiences, but then adapts such that it sends fewer signals thereafter.
Not all sensory receptors are rapidly adapting. Certain pain receptors seem to have little adaptation, or are very slowly adapting, so that as long as the pain stimulus is applied, the person continues to “get the message”! The general rates of adaptation for specific sensory receptors are indicated in the above table.
Pain
Pain and Nociceptors
We have all felt pain, and although uncomfortable, it likely provided us important information about tissue damage — damage that may have gotten worse if pain had not made us aware of the problems at hand. In response to pain we tend to “protect” the damaged tissue from further use and seek appropriate medical attention. Thus pain is a critical sensation for alerting us to problems within the body such that they can be appropriately addressed.
Pain receptors, called nociceptors, are spread throughout most of the body’s tissues, with the exception of the central nervous system. They respond to nociceptive, or noxious, stimuli that lead to our perception of pain. These receptors vary in the specific stimuli that they respond to, as well as how quickly they transmit information to the central nervous system.
You likely realize that there are many noxious stimuli. Extreme temperature, a pinch, blunt impact, cuts, intestinal gas, overuse of joints, and others can all elicit the sensation of pain. This is because all of these stimuli have the ability to either directly activate nociceptors, or cause tissue damage that leads to the release of chemical substances that will activate nociceptors. You also appreciate that the sensation of pain can change over time, where an injury may start out with stinging pain, become dull, and even revert to stinging again under certain conditions, such as when someone touches the injured area. The combination of nociceptors stimulated helps determine the characteristics of the pain that is felt. Examples of nociceptor locations and types are listed in table below.
Location | Nociceptor type | Activated by: |
---|---|---|
Skin | Mechano- | Intense mechanical stimulation |
Chemo- | Many chemical mediators released during tissue damage, including prostaglandins and histamine | |
Thermo- | Mechanical and thermal stimuli | |
Polymodal | High intensity stimuli of various types | |
Silent | Mechanical stimulation after inflammation has set in | |
Viscera | Mechano- | Intense mechanical stimulation |
Thermo- | Mechanical and thermal stimuli | |
Chemo- | Many chemical mediators released during tissue damage, including prostaglandins and histamine | |
Silent | Mechanical stimulation after inflammation has set in | |
Joints | Mechano- | High intensity mechanical stimulation |
Polymodal | Various | |
Silent | Mechanical stimulation after inflammation has set in |
The silent (sleep) nociceptors listed in the table have the unique property that they are normally unresponsive to stimulation until turned “on” by chemicals released during the inflammatory process. This is one reason why, after you have stubbed your toe, or pinched your finger, you may have thought to yourself “this is going to hurt”. You know that once the tissue inflames (swells up), throbbing pain is likely to set in as further nociceptors become activated.
Categories of Pain
Because there are multiple types of nociceptors that can transmit information at different rates, our pain sensation is not always the same. Scientists generally recognize three different pain categories (sensations or stimulus modalities), as described in table below.
Pain Category | Description |
---|---|
Fast pain/pricking pain/sensory pain | Sharp, stinging pain that is well localized. Arises mainly from the skin. |
Burning pain/ soreness pain | Elicited by inflammation secondary to damaged tissue (hit thumb with hammer, etc.). Arises mainly from skin, but also from tissues such as muscle. It is more diffuse and longer in duration than fast pain. |
Aching pain | Poorly localized pain arising from deep structures (joints and viscera). |
Characteristics of Pain
Hyperalgesia and Analgesia
Notice that because chemical and silent nociceptors are activated after tissue injury has set in, our sensations of pain can change over time. An initial cut will activate mechano-nociceptors, sending the fast pain signals along A-delta fibers to the brain. After inflammation in the area has set in, the chemical and/or silent nociceptors may send information along C fibers, producing a different pain sensation. If these silent nociceptors become sensitized by the inflammatory mediators, then we might experience hyperalgesia, where tissue stretch to an injured area can be sensed as a more intense pain.
Our ability to provide analgesia (reduce or block pain) is usually directed at inhibiting the local formation of the mediators that activate chemical nociceptors (this is how aspirin and ibuprofen work), blocking the transmission of signals along peripheral pain fibers (local anesthetics), or interrupting the transmission of pain signals in the CNS (endogenous or exogenous opioids as well as general anesthesia). You may have had experience with a local anesthetic during dental work or when stitches were put in. They act by blocking the ability of nerve fibers to conduct action potentials. Local anesthetics block C fibers more easily, but in time the A-delta fibers are also blocked. This is why the dentist waits awhile after giving the local anesthetic before commencing the work that would otherwise cause pain – you want to make sure your A-delta fibers are fully blocked as well!
CNS Role in Pain – Dermatomes
Although the focus of this section has been on the peripheral sensation and transmission of resulting action potentials, keep in mind that the processing of pain, such as pain sensation, localization and the physical and emotional responses to pain, are functions of the CNS.
Individual sensory neurons that are in proximity to each other are bundled together into nerves that enter the spinal cord through its posterior root or horn. The region of the skin that each spinal nerve carries information from is called its dermatome. Because there are 31 spinal nerves, there are an equal number of dermatomes, each named for the spinal nerve to which it sends information.
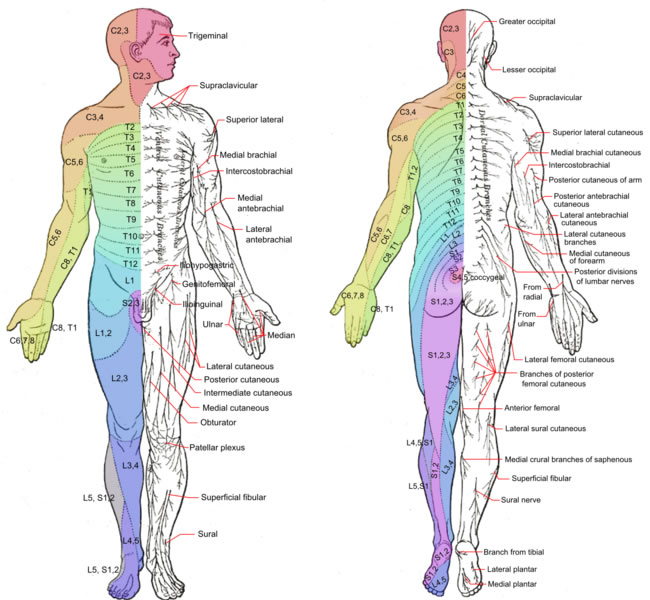
A dermatome map is particularly useful in the diagnosis of spinal cord injuries that interrupt spinal cord transmission. An injured person will lose sensation from all their dermatomes below the level of injury.
Reflexes
So far we have discussed the types and distribution of somesthetic receptors, now let’s consider what the central nervous system might do with the information it is receiving from these receptors. One option is to make conscious decisions about how to react to the information, such as deciding how to change our behavior if we are cold or in pain. As discussed in the section on the autonomic nervous system, another option is to use this sensory information to inform a visceral reflex arc, like those involved in the regulation of blood pressure or body temperature. Here we will consider using sensory information to inform somatic reflexes, where automatic motor responses occur as a result of the sensory stimuli.
No matter which type of CNS mediated reflex or response we are referring to, the general model is the same. Sensory information activates a receptor that sends information to the CNS via an afferent neuron, some level of synaptic or higher level processing occurs, and, if a response is necessary or appropriate, the response is initiated through efferent neurons. You might recognize this as the same model used to maintain homeostasis. Reflexes are a unique category of responses because they do not require the higher centers used for conscious or voluntary responses. Instead reflexes are involuntary, stereotyped (they are repeatable under the same stimulus conditions) responses that occur quickly.
Categories of Reflexes
As mentioned, reflexes can either be visceral or somatic. Visceral reflexes involve a glandular or non-skeletal muscular response carried out in internal organs such as the heart, blood vessels, or structures of the GI tract. In contrast, somatic reflexes involve unconscious skeletal muscle motor responses. Somatic reflexes can either be intrinsic (present at birth) or learned. We will be focusing on intrinsic reflexes, which occur as the result of normal human development. Learned reflexes are much more complicated in their anatomical structure and result from repetitive actions, such as athletic training. Reflexes can also be categorized by the number of synapses they involve (monosynaptic reflex versus polysynaptic reflex) or the relative position of the sensory receptors to the responding muscles (ipsilateral = same side of the body, contralateral = opposite sides of the body).
Stretch Reflex
One of the simplest reflexes is a stretch reflex. In this reflex, when a skeletal muscle is stretched, a muscle spindle in the belly of the muscle is activated. The axon from this receptor travels to the spinal cord where it synapses with the motor neuron controlling the muscle, stimulating it to contract. This is a rapid, monosynaptic, ipsilateral reflex that helps to maintain the length of muscles and contributes to joint stabilization. A common example of this reflex is the knee jerk reflex that is elicited by a rubber hammer striking against the patellar tendon, such as during a physical exam. When the hammer strikes, it stretches the tendon, which pulls on the quadriceps femoris muscle. Because bones and tendons do not typically pull muscles, the muscle “thinks” it is stretching very rapidly, and the reflex acts to counteract this stretch. In doing so, the “knee jerk” occurs.
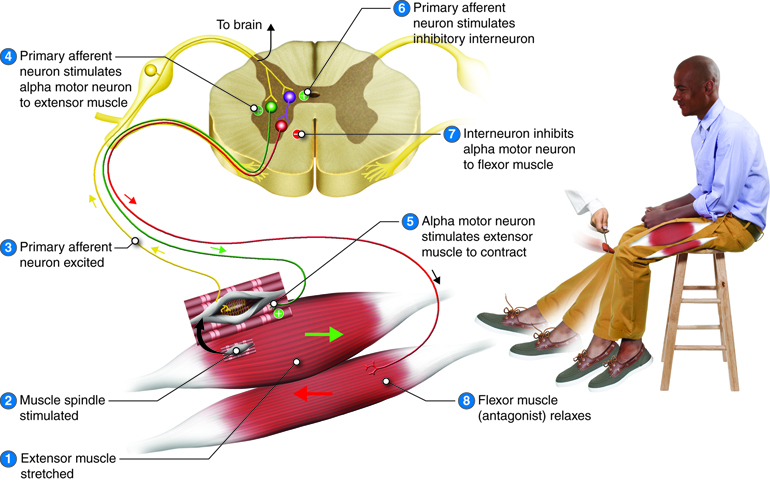
Flexor (Withdrawal) Reflex
Recall from the beginning of this unit that when you touch a hot stove, you reflexively pull your hand away. Sensory receptors in the skin sense extreme temperature and the early signs of tissue damage. To avoid further damage, information travels along the sensory fibers from the skin and into the posterior (dorsal) horn of the spinal cord. Once in the spinal cord, the sensory fibers synapse with a variety of interneurons that mediate the responses of the reflex. These responses included a strong initial withdrawal of the flexor muscle (caused by activation of the alpha motor neurons), inhibition of the extensor muscle (mediated through inhibitory interneurons), and sustained contraction of the flexor (mediated by a spinal cord neuronal circuit). And as already discussed, the sensory information will also travel to the brain to develop a conscious awareness of the situation such that conscious decision-making can take over immediately after the reflex occurs.
Crossed-Extensor Reflex
Imagine what would happen if, when you stepped on a sharp object, it elicited a strong withdrawal reflex of your leg. You would likely topple over. In order to prevent this from happening, as the flexor (withdrawal) reflex involving the injured leg happens, an extension reflex of the opposite (contralateral) leg occurs at the same time, creating a crossed-extensor reflex. In this case, the ipsilateral limb reacts with a withdrawal reflex (stimulating flexor muscles and inhibiting extensor muscles on same side), but the contralateral extensor muscles contract so that the person can appropriately shift balance to the opposite foot during the reflex.
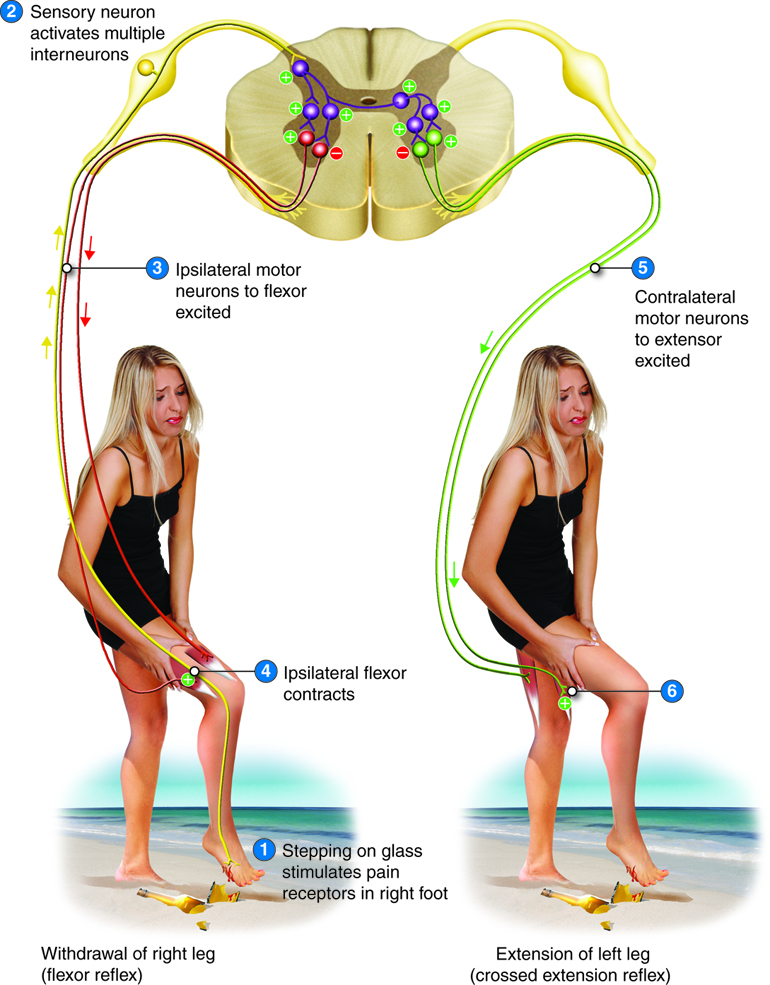
Special Senses: Vision
Anatomy of the Eye
The eyes are located within the skull orbits, which provide protection for the eyes, aswell as provide a place to anchor the soft tissues that support the functions of the eye. The eyelids, with lashes at their leading edges, help to protect the eye from abrasions by blocking particles that may get onto its surface. From the inner surface of each lid, a thin mucous membrane known as the conjunctiva folds in andcovers the surface of the eye. Tears are produced by the lacrimalglands, which are superior and lateral to the orbit in each eye, and they flow over theconjunctiva to wash away particles that may have gotten past the lashes and the lids. Tears flow down through the lacrimal ducts, located on the medialside of each orbit, into the nasal cavity.
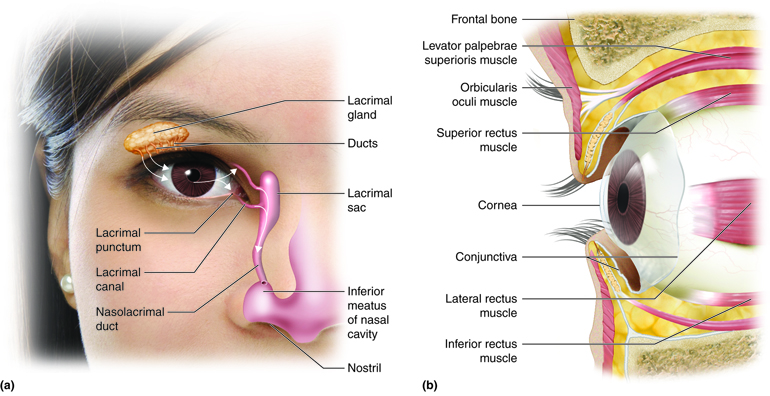
Components of the Eye
The eye itself is a hollow sphere composed of three layers of tissue. The outermost layer is the fibrous tunic which is the white sclera and clear cornea. The two parts of the fibrous tunic are continuous, though they have different properties. The sclera accounts for 5/6 of the surface of the eye, most of which is not visible (though humans are unique in having so much of the “white of the eye” visible). The cornea covers the anterior region of the eye and allows light to pass into the eye where it will eventually stimulate photoreceptors. The next layer of the eye is the vascular tunic, which is mostly composed of the choroid, a highly vascularized connective tissue that provides a blood supply to the adjacent tissue. The choroid is posterior to the ciliary body, a muscular structure that is attached to the lens by the suspensory ligament. The ciliary body focuses light on the back of the eye. Overlaying the ciliary body, and visible in the anterior eye, is the iris, the colored part of the eye that opens in the center as the pupil. The innermost layer of the eye is the neural tunic, which is the retina or the nervous tissue that is responsible for photoreception.
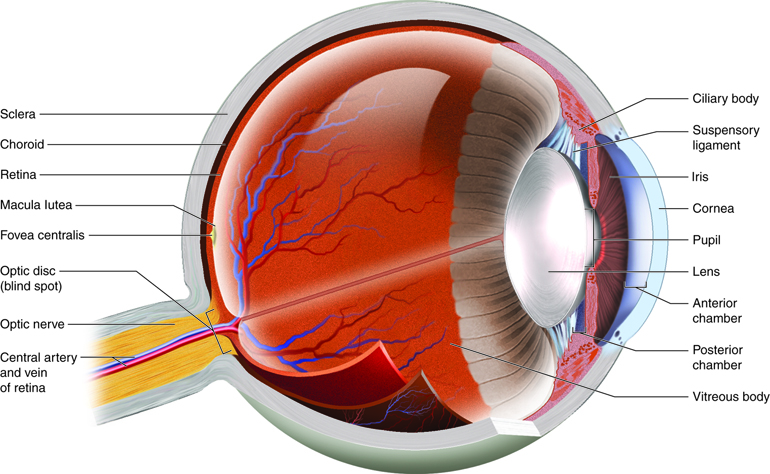
Chambers of the Eye
The eye is also divided into two cavities, the anterior and posterior. The anterior chamber, of anterior cavity, is the space between the cornea and iris. The posterior chamber sits between the iris and the lens. Both the anterior and posterior chambers are filled with a watery fluid called the aqueous humor. The posterior vitreous chamber (also posterior cavity) is posterior to the lens and is filled with a more viscous fluid called the vitreous humor (vitreous body).
Eye Movement
Movement of the eye within the orbit is accomplished by the contraction of six extraocular muscles that originate from the bones of the orbit and insert into the surface of the eye.
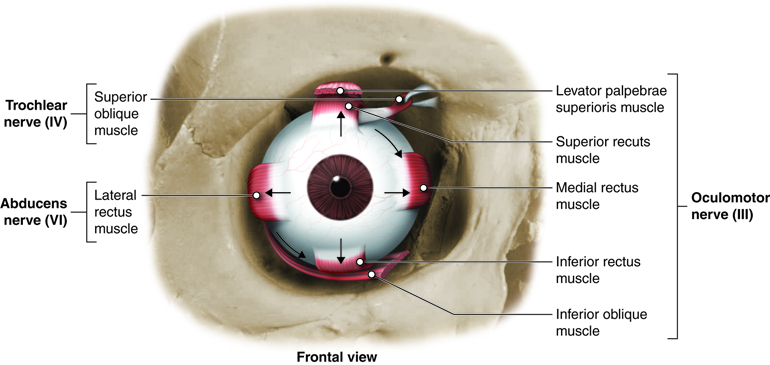
Focusing Light on the Retina
The retina, where the photoreceptors are found, is located at the posterior aspect of the eye. In order for the retina to transmit the most appropriate information to the brain, the light rays must land on the retinal cells in focus and with appropriate intensity. The cornea, pupil (the center of the iris) and the lens are responsible for meeting these requirements.
When light moves from one medium (such as air) into another medium (such as the cornea or lens), any rays not entering at a 90 degree angle will be refracted, or bent. Because both the cornea and lens have curved surfaces, they refract some of the light rays entering the eye. In doing so, they compress the image of what we see so that a large amount of visual information can be processed by a small amount of retinal tissue. The cornea refracts more light than the lens does because its surface is more curved, but the lens has the ability to change its shape, and therefore fine-tune the amount of refraction necessary to focus the light rays on the retina. This process is known as accommodation.
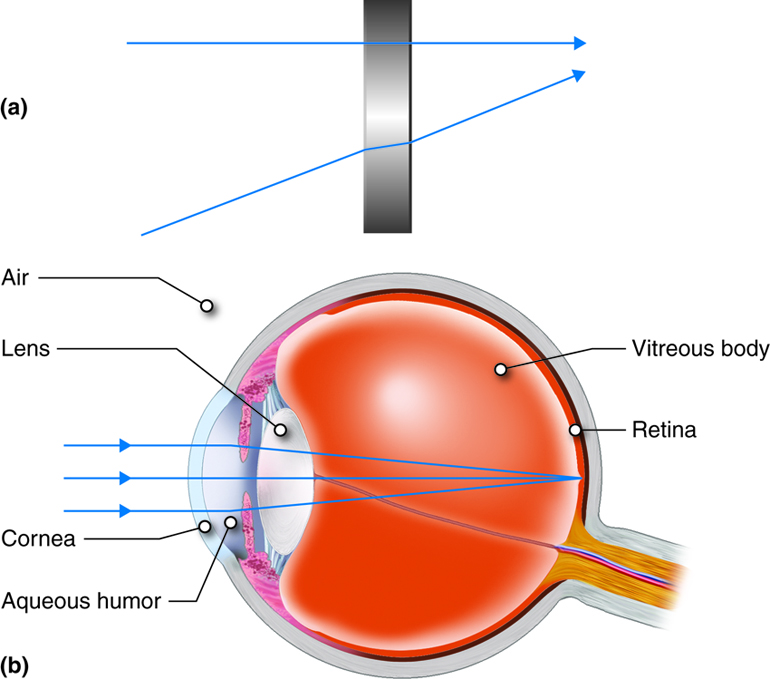
The lens changes its shape in response to changes in tension of the ciliary muscles on the suspensory ligaments (also called zonules) that hold the lens in place. When the ciliary muscles contract, the suspensory ligaments are less taught, causing the lens to become slightly more spherical and refract light more. This is what happens when objects that are being viewed are close, or moved closer. Light coming from objects that are far away do not require as much refraction and are viewed with the ciliary muscles relaxed and more tension on the lens, which makes it more oblong.
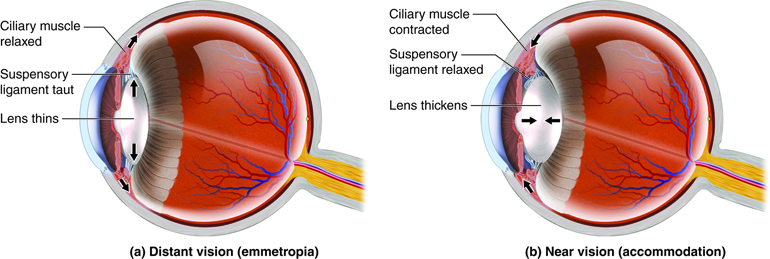
Along with accommodation of the lens when objects are near, the pupil also tends to constrict to allow less peripheral light to enter the posterior chamber of the eye. In doing so, objects can be viewed more crisply. The pupil will also constrict when conditions are bright and dilate under low light conditions. This way the retina can receive an appropriate amount of light to activate its photoreceptors without bleaching them with too much light.
The Retina
The retina is composed of a several layers and contains specialized cells for the initial processing of visual stimuli, with the rest of the visual processing occuring in the central nervous system.
The photoreceptors are found in the retinal layer closest to the back of the eye (outermost layer). When stimulated by light energy, they change their membrane potential and alter the amount of neurotransmitter released onto the bipolar cells. The bipolar cells connect to the retinal ganglion cells (RGC) where amacrine cells also contribute to retinal processing such as contrast enhancement and edge detection. The axons of RGCs, which are lying at the innermost aspect of the retina, collect at the optic disc and leave the eye as the optic nerve. Because of the axons passing through the wall of the eye at the optic disc, there are no photoreceptors resulting in a “blind spot” in the retina. The blind spot in either retina falls in the medial retina and does not process corresponding regions of the visual field.
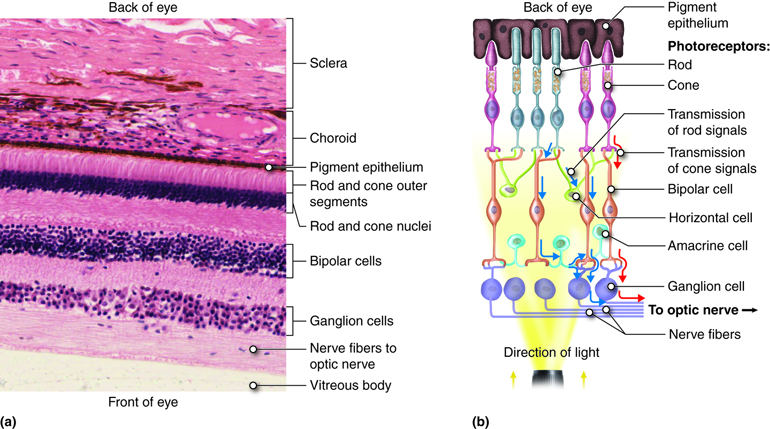
At the exact center of the retina is a point where light is focused by the lens and the greatest visual acuity is found. This is known as the fovea and it is a small dimple in the layers of the retina where there are no blood vessels, ganglion cells or bipolar cells to interrupt light reaching the receptor cells. Because more light passes to the receptor cells at the fovea, it is in this region that visual acuity is the greatest. From this central point of the retina, visual acuity drops off towards the peripheral retina.
Photoreceptor Cells
Light falling on the retina causes chemical changes to pigment molecules (called opsins) in photoreceptors, ultimately leading to a change in the activity of the retinal ganglion cells. Photoreceptor cells have two parts, the inner segment and the outer segment.
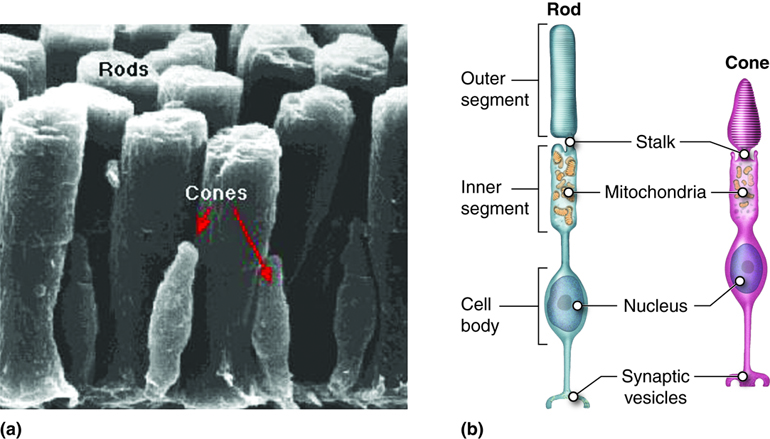
The inner segment contains the nucleus and other common organelles of a cell while the outer segment is a specialized region of the cell where photoreception takes place. There are two types of photoreceptors, rods and cones, based on the shape of their outer segment. The rod-shaped outer segments of rod photoreceptors contain a stack of membrane-bound discs that contain a photosensitive opsin pigment called rhodopsin, which is sensitive to a wide bandwidth of light (white light). The cone-shaped outer segments of cone cells contain one of three photosensitive opsin pigments, called photopsins. Each of the three photopsins are sensitive to a particular bandwidth of light, corresponding to the colors of red, green or blue, allowing for the ability to distinguish color.
Processing Visual Information
The photoreceptors, and other neuronal cells of the retina, send varied types of information to the brain. These include light intensity, colors and the spatial distribution of the information received. All of this information is then carried along the optic nerve and into the optic tract to be distributed to nuclei in the brain. At the point where the optic nerve becomes the optic tract, the optic chiasm is found. At this point, fibers carrying information from the nasal half of the retina on each side decussate (cross over), such that the information from the nasal half of the retina of the left eye crosses over to the right side of the brain and vice versa. In doing so, the left side of the brain receives information from the right visual field of each eye, and the right side of the brain receives information from the left visual field of each eye. This matches the sidedness of the brain to motor control. For example, visual information from the left side of the body, and motor control of the left limbs, are both processed by the right hemisphere of the brain.
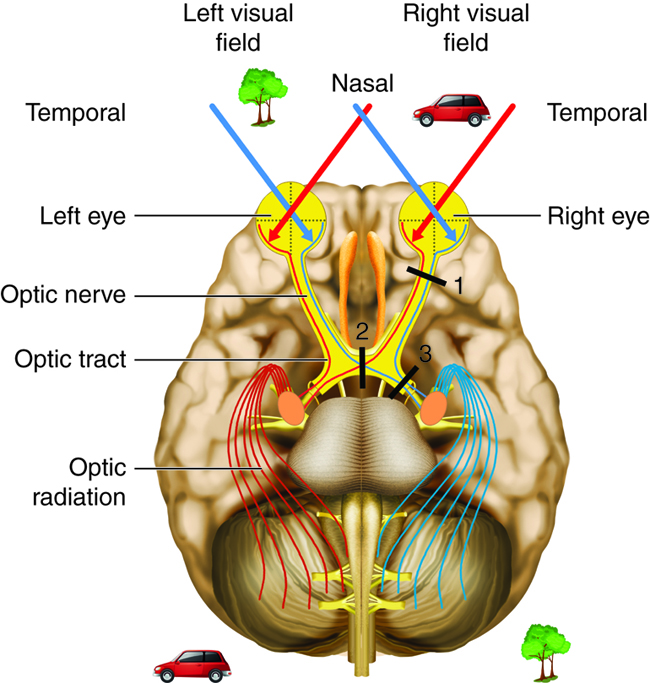
Visual information from the optic tract is sent to a variety of nuclei in the brain. The majority of the visual information flows through the lateral geniculate nucleus of the thalamus into the occipital lobe for perception of vision. From here fibers will carry some information to regions of the parietal and temporal lobes, called the visual association areas. These areas contribute to object recognition (such as recognizing a face) and motion processing (such as catching a moving ball).
Special Senses: Hearing (Audition) and Balance
Structures and Functions of the Outer and Middle Ear
Hearing is the transduction of sound waves into a neural signal that relies on the structures of the ear. The outwardly visible structure that is often referred to as the ear is more correctly referred to as the outer ear (external ear), or the auricle. The C-shaped curves of the auricle direct sound waves towards the ear canal, which enters into the skull through the external auditory meatus of the temporal bone. At the end of the ear canal is the tympanic membrane, or ear drum, which vibrates with the movement of air in sound waves.
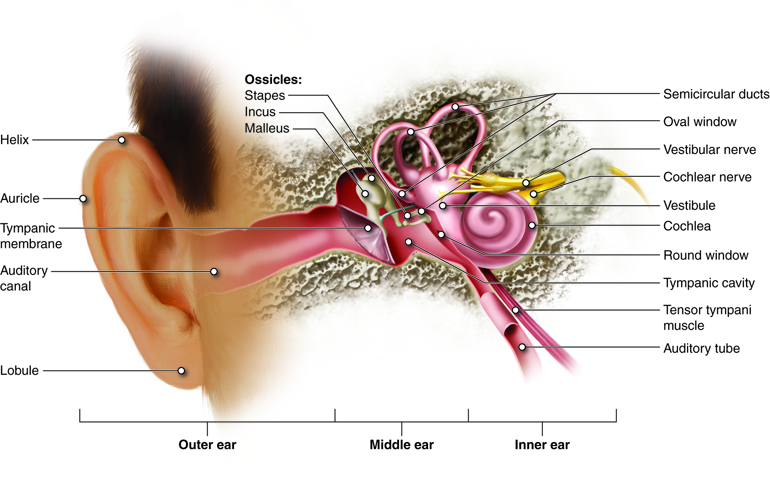
Along the length of the ear canal are ceruminous glands that contribute to the production of cerumen (earwax). Because cerumen is sticky it can help prevent small particles from finding their way to the tympanic membrane. Cerumen also helps prevent bacterial growth, waterproofs the auditory canal and tympanic membrane, and may be a deterrent to small insects.
The middle ear consists of a space spanned by three small bones, the ossicles, which amplify the movements of the tympanic membrane. These small bones are the malleus, incus, and stapes, which are Latin names that roughly translate to hammer, anvil, and stirrup. The malleus is attached to the tympanic membrane and articulates with the incus, which articulates with the stapes. The stapes is then attached to the inner ear where the sound waves will be transduced to a neural signal.
The middle ear is also connected to the pharynx through the auditory tube (Eustachian tube) that helps equilibrate air pressure across the tympanic membrane. When flying, you may have experienced what happens when the pressures across the tympanic membrane are not equal. As the plane climbs, pressure on the outside of the membrane decreases. If there is not a corresponding decrease in pressure in the middle ear, the pressure difference will cause the eardrum to push outward, causing pain and muffled hearing. The auditory tube is normally closed, but will typically open when muscles of the pharynx contract during swallowing or yawning. For this reason, chewing gum or drinking as the plane climbs will often relieve these symptoms. The auditory tube also provides a pathway of drainage for fluids that accumulate during middle ear infections (otitis media). Unfortunately, it is also the auditory tubes that play a role in causing otitis media, as microorganisms can use this path to move from the pharynx into the middle ear. This is especially common in children.
Structure and Function of the Inner Ear
The inner ear is entirely enclosed within the temporal bone. It has two separate regions, the cochlea and vestibule, which are responsible for hearing and balance, respectively. The neural signals from the two regions of the inner ear are relayed to the brainstem through separate fiber bundles, but which run together as the vestibulocochlear nerve.
Sound information is transmitted from the middle ear to the inner ear via the stapes attachment to the oval window, which is a membrane at the beginning of the cochlea. As the tympanic membrane vibrates from sound waves, the ossicles amplify that vibration, and then the oval window moves with the same vibrations. The oval window is at the beginning of a tube that runs the length of the cochlea to its tip (helicotrema) and back alongside itself to end at another membrane called the round window (secondary tympanic membrane).
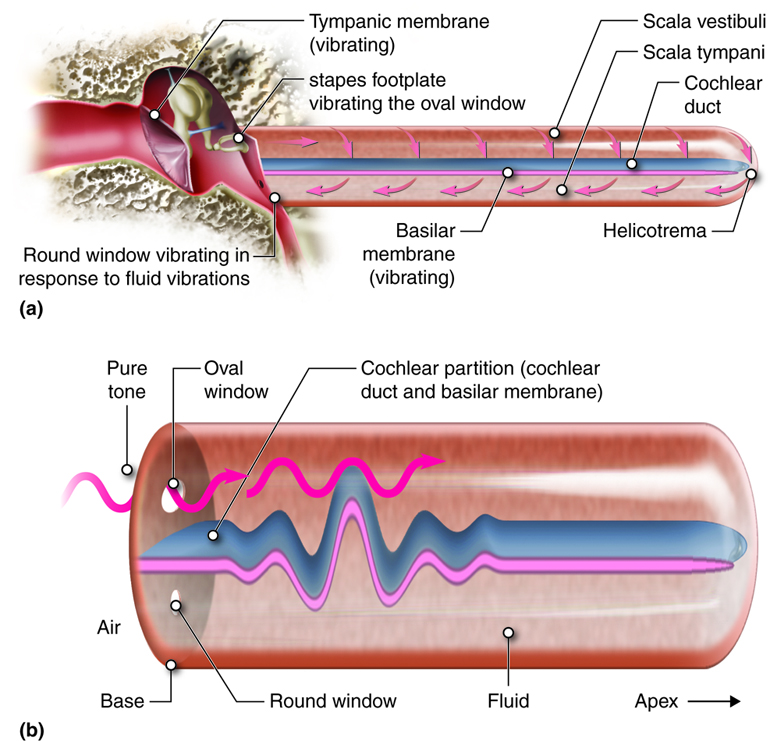
As the oval window is pushed in by sound waves, fluid within this tube is pushed along its length and the round window at its other end can bulge out as a result of that movement. Likewise, when the oval window is pulled back, the fluid inside this tube is drawn back and the round window can pucker in to compensate. As vibrations of the tympanic membrane are transmitted through the ossicles, a wave (often referred to as standing wave because of its properties) is created within the fluid in the cochlea that displaces sections of the cochlear partition (cochlear duct and basilar membrane). It is these waves that are detected by the sensing cells found attached to the basilar membrane.
The tube running from the oval to the round window in the cochlea is separated into two spaces. From the oval window to the tip of the cochlea the tube is referred to as the scala vestibuli and from the tip of the cochlea back to the round window it is the scala tympani. These spaces can be seen in a cross-section of one turn of the cochlea.
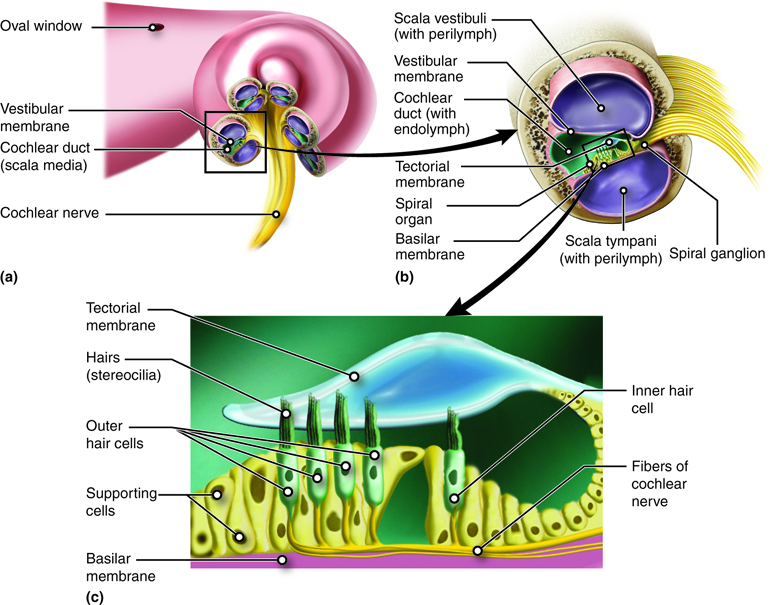
The two spaces are on either side of the cochlear duct, which is the space that contains the structures that transduce sound into the neural signal. Those structures are contained within the spiral organ or organ of Corti, which lies on top of the basilar membrane that separates it from the scala tympani. The spiral organ contains hair cells with stereocilia on their apical membrane. The stereocilia bend in response to movement of the basilar membrane relative to the partially fixed tectorial membrane. Depending on which direction the stereocilia bend, they open or close ion channels, leading to signal changes in the cochlear nerve.
The cochlea encodes auditory stimuli based on frequency between 20 Hz and 20,000 Hz, the range of human hearing. Higher frequencies (higher pitch) cause the basilar membrane close to the base of the cochlea to move and lower frequencies (lower pitch) cause the basilar membrane closer to the tip of the cochlea to move. Loudness, or sound intensity, is determined by the number of hair cells in an area that are stimulated. A louder sound produces greater displacement of the basilar membrane, leading to a greater number of stereocilia responding.
The information sensed by the spiral organ of the cochlea is transmitted into the brain via the vestibulocochlear nerve (cranial nerve VIII) to nuclei in the pons. At the level of the pons and related connections in the midbrain, some processing of auditory information occurs, including identifying where a sound is coming from and responding to loud noises. From the pons and midbrain, fibers carrying auditory information project to the thalamus and then, from there to the primary auditory cortex of the temporal lobe. It is here that the conscious perception of sound is located.
Dynamic Equilibrium
Along with hearing, the inner ear is responsible for encoding information about equilibrium (the sense of balance), which it does in the vestibular apparatus.
Similar to the cochlea, the vestibular structures use hair cells with stereocilia to detect movement of fluid, in this case, in response to changes in head position or acceleration. Detection of head position when the body is stationary is termed static equilibrium. The information for static equilibrium comes from the utricle and saccule. Dynamic equilibrium is the perception of acceleration. Information for dynamic equilibrium can come from the utricle and saccule, which detect linear acceleration, and/or the semicircular canals, which detect angular acceleration. The neural signals generated from the vestibule are transmitted to the brainstem and cerebellum from sensory neurons in the vestibular ganglion.
The saccule and utricle each contain a sense organ, called the macula, where stereocilia and their supporting cells are found. These maculae (plural) are oriented 90 degrees to one another so that they respond to positions in different planes.

At the base of each semicircular canal, where it meets with the vestibule is an enlarged region known as the ampulla, which contains a hair-cell containing structure, called the crista ampullaris that responds to rotational movement. The stereocilia of the hair cells extend into the cupula, a membrane that attaches to the top of the ampulla.
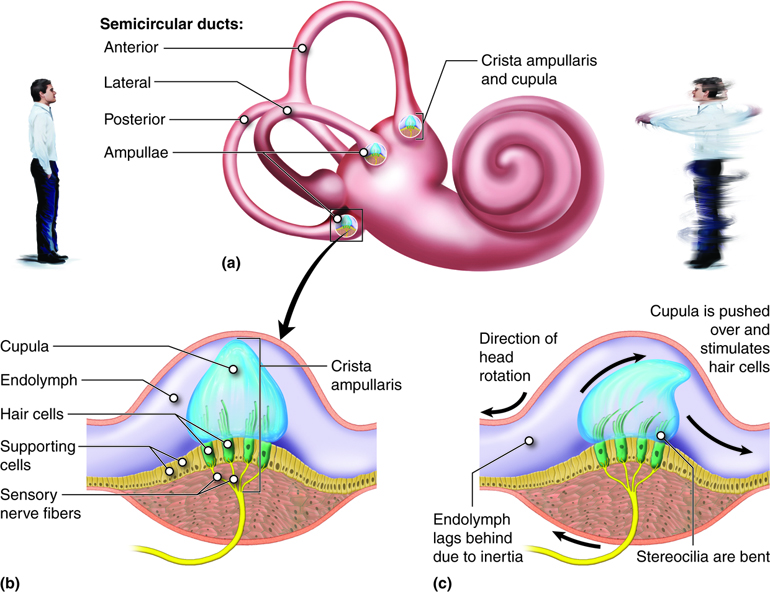
Vestibular Nerve Impulses
Information from the vestibular apparatus projects to a wide range of structures in the brain. The information enters the brain via the vestibulocochlear nerve (cranial nerve VIII) where it synapses at vestibular nuclei in the pons and medulla. From here, information travels to a number of other regions to stimulate reflexes and develop our conscious awareness of position and movement.
Special Senses: Taste (Gustation)
Taste, or gustation, is a sense that develops through the interaction of dissolved molecules with taste buds. Currently five sub-modalities (tastes) are recognized, including sweet, salty, bitter, sour, and umami (savory taste or the taste of protein). Umami is the most recent taste sensation described, gaining acceptance in the 1980s. Further research has the potential to discover more sub-modalities in this area, with some scientists suggesting that a taste receptor for fats is likely.
Taste is associated mainly with the tongue, although there are taste (gustatory) receptors on the palate and epiglottis as well. The surface of the tongue, along with the rest of the oral cavity, is lined by a stratified squamous epithelium. In the surface of the tongue are raised bumps, called papilla, that contain the taste buds. There are three types of papilla, based on their appearance: vallate, foliate, and fungiform.
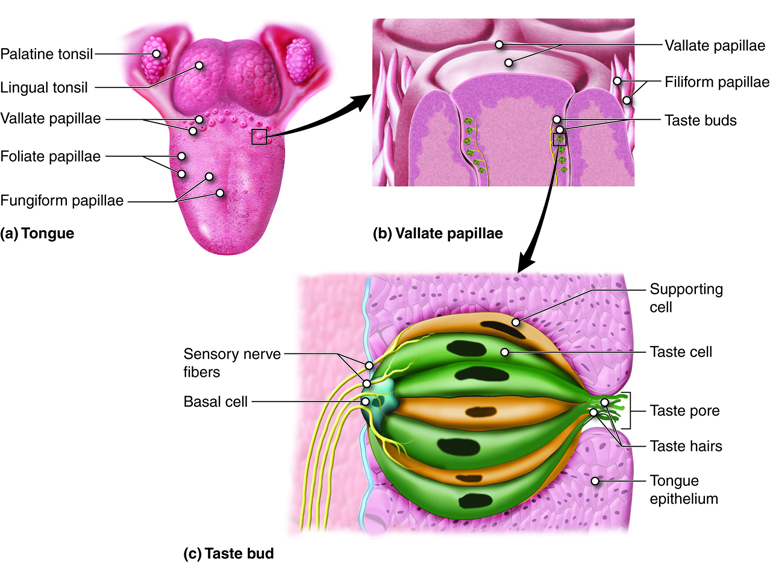
The number of taste buds within papillae varies, with each bud containing several specialized taste cells (gustatory receptor cells) for the transduction of taste stimuli. These receptor cells release neurotransmitters when certain chemicals in ingested substances (such as food) are carried to their surface in saliva. Neurotransmitter from the gustatory cells can activate the sensory neurons in the facial and glossopharyngeal cranial nerves.
Primary Taste Sensations
As previously mentioned, five different taste sensations are currently recognized. The first, salty, is simply the sense of Na+ concentration in the saliva. As the Na+ concentration becomes high outside the taste cells, a strong concentration gradient drives their diffusion into the cells. This depolarizes the cells, leading them to release neurotransmitter.
The sour taste is transduced similar to that of salty, except that it is a response to the H+ concentration released from acidic substances (those with low pH), instead of a response to Na+. For example, orange juice, which contains citric acid, will taste sour because it has a pH value of about 3. Of course, it is often sweetened so that the sour taste is masked. As the concentration of the hydrogen ions increases because of ingesting acidic compounds, the depolarization of specific taste cells increases.
The other three tastes; sweet, bitter and umami are transduced through G-protein coupled cell surface receptors instead of the direct diffusion of ions like we discussed with salty and sour. The sweet taste is the sensitivity of taste cells to the presence of glucose dissolved in the saliva. Molecules that are similar in structure to glucose will have a similar effect on the sensation of sweetness. Other monosaccharides such as fructose or artificial sweeteners like aspartame (Nutrasweet™), saccharine, or sucralose (Splenda™) will activate the sweet receptors as well. The affinity for each of these molecules varies, and some will taste “sweeter” than glucose because they bind to the G-protein coupled receptor differently.
The bitter taste can be stimulated by a large number of molecules collectively known as alkaloids. Alkaloids are essentially the opposite of acids, they contain basic (in the sense of pH) nitrogen atoms within their structures. Most alkaloids originate from plant sources, with common examples being hops (in beer), tannins (in wine), tea, aspirin, and similar molecules. Coffee contains alkaloids and is slightly acidic, with the alkaloids contributing the bitter taste to coffee. When enough alkaloids are contained in a substance it can stimulate the gag reflex. This is a protective mechanism because alkaloids are often produced by plants as a toxin to deter infectious microorganisms and plant eating animals. Such molecules may be toxic to animals as well, so we tend to avoid eating bitter foods. When we do eat bitter foods, they are often combined with a sweet component to make them more palatable (cream and sugar in coffee, for example).
The taste known as umami is often referred to as the savory taste. The name was created by the Japanese researcher who originally described it. Like sweet and bitter, it is based on the activation of G-protein coupled receptors, in this case by amino acids, especially glutamine. Thus, umami might be considered the taste of proteins, and is most associated with meat containing dishes.
Gustatory Nerve Impulses
Once the taste cells are activated by molecules liberated from the things we ingest, they release neurotransmitters onto the dendrites of sensory neurons. These neurons are part of the facial and glossopharyngeal cranial nerves, as well as a component within the vagus nerve dedicated to the gag reflex. The facial nerve connects to taste buds in the anterior third of the tongue. The glossopharyngeal nerve connects to taste buds in the posterior two thirds of the tongue. The vagus nerve connects to taste buds in the extreme posterior of the tongue, verging on the pharynx, which are more sensitive to noxious stimuli like bitterness.
Axons from the three cranial nerves carrying taste information travel to the medulla. From there much of the information is carried to the thalamus and then routed to the primary gustatory cortex, located near the inferior margin of the post-central gyrus. It is the primary gustatory cortex that is responsible for our sensations of taste. And, although this region receives significant input from taste buds, it is likely that it also receives information about the smell and texture of food, all contributing to our overall taste experience. The nuclei in the medulla also send projections to the hypothalamus and amygdalae, which are involved in autonomic reflexes such as gagging and salivation.
Special Senses: Smell (Olfaction)
The other special sense responsive to chemical stimuli is the sense of the smell, or olfaction. The olfactory receptor neurons are incorporated into a limited region of the nasal epithelium in the superior nasal cavity.
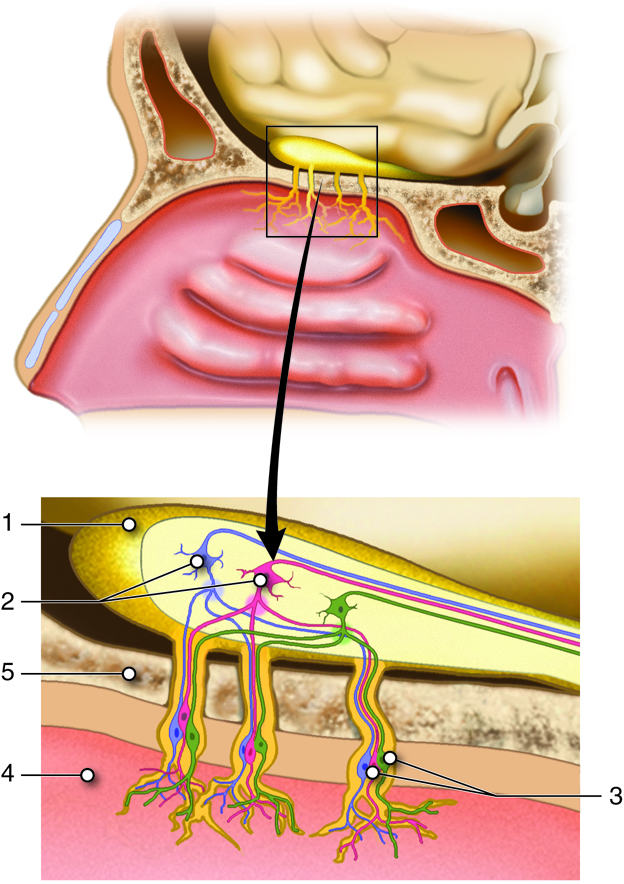
This region is referred to as the olfactory epithelium and contains bipolar sensory neurons with dendrites extending from the apical surface of the epithelium into the mucus lining the nasal cavity. As airborne molecules are inhaled through the nose, they pass over the olfactory epithelium and dissolve into the mucus. The odorant molecules bind to proteins that keep them dissolved in the mucus and help transport them to the olfactory dendrites. The odorant-protein complex binds to a receptor protein on the membrane of the olfactory cell. The olfactory odorant receptors are G-protein coupled receptors that will cause a transient depolarization in membrane potential that will lead to an action potential if the stimulus is strong enough.
Olfactory Nerve Impulses
The axons of the olfactory neurons extend from the basal surface of the epithelium, through an olfactory foramen in the cribriform plate of the ethmoid bone, and into the olfactory bulb, located on the ventral surface of the frontal lobe. Collectively the axons that pass through the cribriform plate are called the olfactory nerve. From the olfactory bulb, axons project to structures within the limbic cortex, including the cortex in the temporal lobe associated with long-term memory formation.

Smell is the one sensory modality that does not require a synaptic connection in the thalamus before connecting to the cerebral cortex. Smell can often be a potent trigger for memories because of this intimate connection of the olfactory system with the cerebral cortex. It can also trigger visceral reflexes through connections within the reticular formation. For example, some people will vomit at the smell of another person’s vomit.
Because of the noxious chemicals that are inhaled into the nasal cavity, these cells need to be replaced on a regular basis. The nasal epithelium, including the olfactory cells, can be harmed by airborne toxic chemicals. To reduce the risk of olfactory neurons being harmed by these toxins, they have a limited lifespan of about 60 days. Because of this imposed cell death, stem cells within the nasal epithelial layer differentiate into new olfactory cells. In doing so, axons of the new neurons have to find their way to the targets in the olfactory bulb. They are able to do this by growing along the axons that are already in place in the cranial nerve. This is the only place in the body where we continually make new neuronal cells.
Olfactory System Dysfunctions
If any event, such as blunt force trauma of a car accident, leads to the loss of the olfactory nerve, the sense of smell can be lost. This condition is known as anosmia. One way this condition can develop is if the brain moves relative to the ethmoid bone, which is likely to shear (tear) the axons of the olfactory cells. Professional fighters can have anosmia as a result repeated trauma to the head. Certain pharmaceuticals, such as antibiotics, can cause anosmia because they can kill off all the olfactory neurons at once. If no axons are in place within the olfactory nerve, then the axons cannot grow back into the olfactory bulb leaving the person with a long-term loss of smell. There are temporary causes of anosmia, as well, usually based on inflammatory responses in relation to infection or allergy.
You have probably noticed that when you have a cold, the associated loss of smell usually results in food tasting bland. Since taste is comprised of only a small number of sensations, we use the olfactory system to recognize differences between many foods, especially in terms of the spices used. If we lack the numerous smells to enrich the taste experience, we often add extra seasonings to further stimulate the tongue.
The ability of olfactory neurons to be replaced by stem cells can be lost with age leading to age-related anosmia. When this affects an elderly person, they often turn to using increased salt on their food. Unfortunately, increased sodium intake also leads to an increase in blood volume and blood pressure.
Nervous Sensory Functions Review
Stimuli in the environment activate specialized receptors in the peripheral nervous system. The classification of receptors into types can be based on three different criteria: structure of the receptors, location of the receptors relative to the stimuli they sense, and by the types of stimuli to which they respond. Regardless of type, the function of these receptors is to transduce a stimulus from one form of energy (chemical, physical, etc.) into a change in the cell membrane potential that may or may not create an action potential. Collectively, the receptors and associated neurons that sense and process information related to our somesthetic senses are called the somatosensory system. Make sure you understand the different categories of receptors within this system.
Consider again the learning objectives for this module. Could you demonstrate each of these objectives? If not, consider reviewing content related to these objectives.
- Classify receptors based on structure, location relative to the stimulus, and types of signals they transduce.
- Explain the distribution of receptors involved in providing information for our general (somesthetic) senses.
- Describe the types of information (modality) detected by the receptors associated with the somesthetic senses and the phenomenon of adaptation.
- Explain pain function, nociceptor distribution, and distinguish the fiber types that carry their signals.
- Describe pain in terms of hyperalgesia, analgesia, and receptive field.
- Define dermatome and explain how dermatomes can be used in neurological exams for diagnosing nerve damage.
- Describe reflexes, reflex responses, and distinguish types of reflexes.
- Describe the structures and functions of the eye.
- Explain how the path of light through the eye causes vision.
- Trace the path of nerve impulses from the retina to various parts of the brain.
- Identify the hearing structures of the outer, middle and inner ear and describe their functions.
- Describe the path of nerve impulses from the ear to various parts of the brain.
- Distinguish between static and dynamic equilibrium, describe the structures involved, and their functions.
- Explain the gustation and describe the structures involved.
- Explain how odorants activate olfactory receptors.
- Predict the types of problems that would occur in the body if the olfactory system was not functioning normally.